the Creative Commons Attribution 4.0 License.
the Creative Commons Attribution 4.0 License.
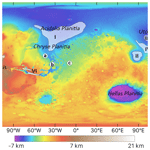
An overview of sedimentary volcanism on Mars
Dorothy Oehler
Adriano Mazzini
Ernst Hauber
Goro Komatsu
Giuseppe Etiope
Vojtěch Cuřín
Extensive fields of sub-kilometre- to kilometre-scale mounds, cones, domes, shields, and flow-like edifices cover large parts of the martian lowlands. These features have been compared to structures on Earth produced by sedimentary volcanism – a process that involves subsurface sediment/fluid mobilisation and commonly releases methane to the atmosphere. It was proposed that such processes might help to explain the presence of methane in the martian atmosphere and may also have produced habitable, subsurface settings of potential astrobiological relevance. However, it remains unclear if sedimentary volcanism on Earth and Mars share genetic similarities and hence if methane or other gases were released on Mars during this process. The aim of this review is to summarise the current knowledge about mud-volcano-like structures on Mars, address the critical aspects of this process, identify key open questions, and point to areas where further research is needed to understand this phenomenon and its importance for the Red Planet's geological evolution. We show here that after several decades of exploration, the amount of evidence supporting martian sedimentary volcanism has increased significantly, but as the critical ground truth is still lacking, alternative explanations cannot be ruled out. We also highlight that the lower gravity and temperatures on Mars compared to Earth control the dynamics of clastic eruptions and surface emplacement mechanisms and the resulting morphologies of erupted material. This implies that shapes and triggering mechanisms of mud-volcano-like structures may be different from those observed on Earth. Therefore, comparative studies should be done with caution. To provide a better understanding of the significance of these abundant features on Mars, we argue for follow-up studies targeting putative sedimentary volcanic features identified on the planet's surface and, if possible, for in situ investigations by landed missions such as that by the Zhurong rover.
- Article
(12421 KB) - Full-text XML
-
Supplement
(291 KB) - BibTeX
- EndNote
The buoyant ascent of liquefied, fluid-rich, and fine-grained sediments through a lithologic succession and its subsequent intrusion or extrusion (hereafter referred to as mud volcanism, MV; Kopf, 2002) is a common phenomenon on Earth. Also known as subsurface sediment and fluid mobilisation (van Rensbergen et al., 2003), it is observed in sedimentary basins typically characterised by the rapid accumulation of fine-grained and organic-rich deposits (Mazzini and Etiope, 2017, and references therein). Images of the martian surface acquired in the early 1970s by the Mariner 9 and Viking Orbiter missions revealed the existence of large outflow channels likely incised by flooding events capable of transporting large amount of sediments (e.g. Baker and Milton, 1974; Komatsu and Baker, 1997) that were subsequently deposited in giant impact basins acting as local depocentres (Lucchitta et al., 1986). Despite these observations, MV was never considered as a process that could have shaped the surface of Mars. Only a few early works hypothesised this type of activity based on low-resolution imagery at specific localities where pitted cones, mounds, and/or associated flows have been observed (e.g. Davis and Tanaka, 1995; Tanaka, 1997; Ori et al., 2000, 2001). A renewed interest for subsurface sediment mobilisation processes emerged when higher-resolution images became available through the Mars Global Surveyor (MGS), Mars Express (MEX), and Mars Reconnaissance Orbiter (MRO) missions and, importantly, some later studies reporting the detection of methane in the martian atmosphere (Krasnopolsky et al., 2004; Formisano et al., 2004; Mumma et al., 2009; Webster et al., 2018). On Earth, MV is an important source of methane released to the atmosphere (e.g. Dimitrov, 2003; Milkov et al., 2003; Etiope and Milkov, 2004). By analogy it was then hypothesised that gas released at mud-volcano-like features could be responsible for the atmospheric methane observed on Mars (e.g. Skinner and Mazzini, 2009; Komatsu et al., 2011; Etiope et al., 2011; Oehler and Etiope, 2017). Although today the existence of methane on Mars is debated (e.g. Oehler and Etiope, 2021; Grenfell et al., 2022) after the ExoMars Trace Gas Orbiter (TGO) failed to detect it (Korablev et al., 2019; Knutsen et al., 2021), interest in mud-volcano-like structures on Mars remains high. This geological phenomenon may have an important role in atmospheric gas emissions. Additionally, the eruption of deeply buried deposits at putative clastic eruption sites provides a window into the subsurface and the sedimentary history of Mars, which is otherwise only provided by impact crater excavation (e.g. crater central uplifts; Cockell and Barlow, 2002; Mustard et al., 2009; Quantin et al., 2012). The source region of remobilised sediments and fluids may have been located in habitable deep environments (e.g., Michalski et al., 2013; Stamenković et al., 2021; see the review by Cockell, 2014, for limitations of subsurface habitat niches). Therefore, erupted materials represent prime targets for in situ investigations and the search for biosignatures (Westall et al., 2015).
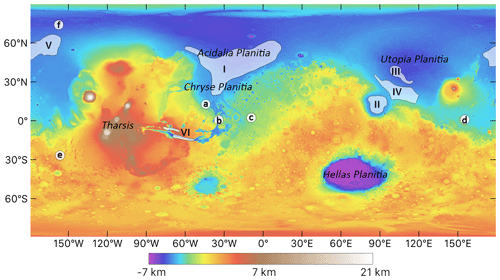
Figure 1Global Mars Orbiter Laser Altimeter (MOLA) topographic map showing the positions of the main fields of martian mud-volcano-like structures. Letters refer to local evidence of sedimentary volcanism in (a) Simud and Tiu valles, (b) Hydraotes Chaos, (c) Firsoff crater, (d) Medusae Fossae Formation, (e) unnamed flat-floored basin in Terra Sirenum, (f) Scandia Colles; roman numerals refer to regional evidence in (I) Chryse and Acidalia planitiae, (II) Isidis Planitia, (III) Adamas Labyrinthus, (IV) Amenthes/Nepenthes region, (V) Arcadia Planitia, and (VI) Candor and Coprates chasmata. See the main text and table for full description of the evidence. MOLA Science Team.
The first hints at possible mud-volcano-like structures on Mars were provided by Viking Orbiter images, which revealed the presence of dozens to thousands of small pitted cones on the floors of large ancient impact basins (e.g. Allen, 1979; Frey et al., 1979). After several decades of Mars exploration, the amount of evidence supporting sedimentary volcanism has significantly increased (Fig. 1). The aim of this review is to summarise current knowledge, identify key open questions, and point to areas where further research is needed to understand this phenomenon and its importance for Mars' geological evolution. Section 2 provides the definition of MV and reviews its main characteristics observed on Earth. Here, we also identify some key requirements needed to generate terrestrial MV and, by analogy, some key questions that should be addressed when searching for evidence to validate the presence of possible subsurface sediment mobilisation on the Red Planet. In Sect. 3, we describe the morphological and morphometrical properties of potential mud-volcano-like surface structures and show some typical examples of the different morphological classes, highlighting the similarities to and differences from terrestrial mud volcanoes. Section 3.4 defines the specific martian environmental conditions and their influence on potential sedimentary volcanic processes. Section 4 assesses the prerequisites and the possible timing of the occurrence of sedimentary volcanism during martian history. In Sect. 5, we discuss putative sedimentary volcanism in the context of Mars' geologic evolution and its implications for habitability. Finally, in Sect. 6 we also suggest measurements by present and future missions (including promising sampling locations) as well as laboratory experiments and modelling activities to test the sedimentary volcanism hypothesis on Mars.
Finally, we note that the authors of the paper have different opinions and views about the subject of this paper. So several aspects addressed in the work are not unanimously agreed upon, and therefore the paper represents an arena of debate, rather than a consensual overview.
In this section, we briefly illustrate the main features of surface sedimentary mud-fluid manifestations observed on Earth, which include sedimentary volcanoes (or mud volcanoes) and some variants. We also emphasise the issue of the potentially ambiguous identification of the various surface manifestations and of the terminology reported in the literature. A correct definition of the process and related surface manifestations is essential for studying and understanding the putative sedimentary volcanism and mud-volcano-like structures identified on Mars. Once the minimum requirements, conditions, and factors necessary to generate terrestrial mud volcanoes are defined, by analogy the same prerequisite can be inferred for the martian structures discussed in this work, assuming these are truly a product of sedimentary volcanism. More specifically, we will try to answer two key questions for determining whether the mud-volcano-like structures on Mars may have environmental and biological implications similar to those of terrestrial mud volcanoes: (a) does the surface mud always stem from a deep shale mobilised (diapir) along a fault? and (b) is the presence of gas necessary in sedimentary volcanism? Is that gas mostly methane, like on Earth? If the answers are yes, the martian mud-volcano-like structures are very likely sites where biomarkers can be better preserved and are, or have been, sites of methane release to the martian atmosphere.
2.1 Sedimentary volcanism: definitions and genetic process
Terrestrial sedimentary volcanoes are widely studied from geological, geophysical, and geochemical points of view; for details on their geographic distribution, inventories, characteristics, and impact on the environment, atmosphere, and energy resource exploration, we primarily refer the reader to the main review works and references therein (e.g. Dimitrov, 2002; Kopf, 2002; Mazzini and Etiope, 2017).
However, it is crucial to clarify that not all muddy-fluid manifestations are mud volcanoes. Natural gas and petroleum geologists agree that the term “mud volcano” should not be used for any gas manifestation resembling a mud pool or where extrusive mud gives rise to small conic edifices (e.g. Kopf, 2002; Mazzini and Etiope, 2017). Many CO2 vents, related to geothermal or hydrothermal environments, may show such characteristics. It is not only a problem of semantics because the attribution of “mud volcano” to a surface gas manifestation implies the existence of a series of specific geologic processes and features.
The definition of mud volcano is strictly linked to the genetic mechanisms that are essential to generate this specific geological phenomenon. The processes are schematically illustrated in Fig. 2. Basically, a mud volcano is characterised by the surface release of mostly deep-seated shale deposits that are brecciated and transported along a fracture system or fault (shale is for a mud volcano what magma is for an igneous volcano). The shale and other rock types are fractured and mobilised, in a diapir style, due to the combination of three factors: (a) lateral tectonic compression; (b) gravitational instability of shale, i.e. typically when shale is a low density sediment underlying another sedimentary rock with higher density; and (c) overpressure of gas and water from an underlying source. This source is generally a gas reservoir, which provides the necessary pressure, likely the factor triggering the mobilisation of the shale (Mazzini and Etiope, 2017). The erupted sediments are defined with the term “mud breccia” and consist of a mix of comminuted and brecciated sediments and rocks that originate from various units and formations intersected by the conduit. Two components are typically identified in the mud breccia: (a) a fine-grained matrix (consisting mostly of clay and fine sediments in large part originating from the low density shales) and (b) a set of rock clasts with sizes ranging from a few cubic centimetres to some cubic metres, stripped from the various sedimentary formations that have been pierced by the diapir. The depth of the reservoirs (which can be found by seismic imaging and/or drilling) and the origin of the clasts are fundamental to assessing the minimum depth of the mud volcano system. Mud volcanoes are located almost exclusively in convergent basins but they can occur along any type of fault, with normal, reverse, or strike-slip kinematics (Ciotoli et al., 2020).
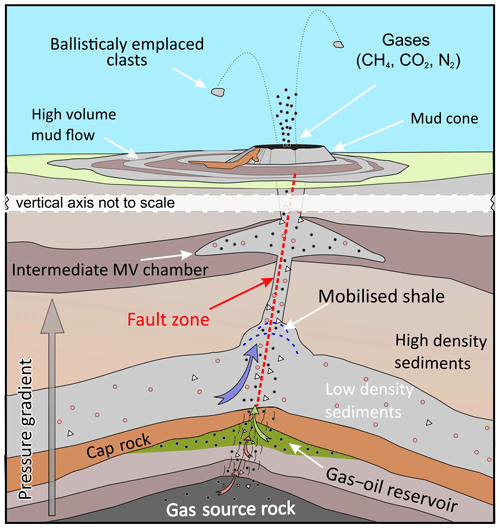
Figure 2A conceptual drawing illustrating the main elements associated with MV on Earth both on the surface and in the subsurface. Red dashed line represents fault zone and different colours for arrows mark various sources of liquids. Figure is adapted from Mazzini and Etiope (2017).
Sedimentary volcanism on Earth is exclusively observed in petroleum-bearing sedimentary basins (see Mazzini and Etiope, 2017, and references therein), i.e. in areas with gas–oil systems (source rocks, reservoirs, and generally in faulted anticlines). Therefore, the erupted gas is mainly methane, associated with other hydrocarbon gases in trace amounts (ethane, propane, and butane) and non-hydrocarbon gases typically occurring in sedimentary reservoirs (CO2, N2, minor amounts of He, and H2S). Methane is mostly of deep thermogenic origin, but often including shallower components of secondary microbial methane (Etiope et al., 2009). A few mud volcanoes in petroliferous basins may release more N2, due to differential solubility in uplifted basins, which also induces higher helium concentrations (Etiope et al., 2011). Water is typically salty and enriched in various elements, largely sourced from the brines accumulated in the gas–oil reservoir (fossil water) in addition to connate water (trapped in sediments), which may also derive from the illitisation of clayey minerals (smectite–illite transformation; Mazzini and Etiope, 2017). Meteoric water can also mix with the fossil water during its upwelling.
2.2 Variants
The mud volcano system depicted in Fig. 2 may be more complex and a few variants of this classic scheme may exist. In some cases, the gas may stem from multilayer reservoirs and the mobilised shales may originate from different sedimentary units and, likewise, multiple source rocks may be involved in the plumbing system (e.g. Guliyev and Feizullayev, 1997; Inan et al., 1997; Cooper, 2001). In addition, a set of other surface sedimentary mud-fluid manifestations exists on Earth that may have sizes and morphologies that resemble those of mud volcanoes. For this reason, the attribution of sedimentary volcanism for these variants may be uncertain, ambiguous, or susceptible to subjective and/or misleading interpretations. However, more detailed studies reveal that these structures have genetic mechanisms, and accordingly names, that are different from those of sedimentary volcanism, with examples given below.
- a.
Sediment-hosted geothermal systems. Some sedimentary basins, featuring petroleum systems, developed above deep geothermal systems (e.g. along grabens) and can be associated with igneous intrusions, deep magmatic chambers, or lateral migration of hydrothermal fluids. These systems can be characterised by elevated CO2 and H2O pressures. These settings are referred to as sediment-hosted geothermal systems (SHGSs), where CO2-dominated fluids migrate upward and mix with the gaseous hydrocarbons hosted in the shallower sedimentary formations (i.e. Procesi et al., 2019). SHGSs are essentially hybrid systems and the relative fraction of the two endmembers (sedimentary CH4 and geothermal CO2, with related waters) may vary greatly. By definition, however, a SHGS releases on the surface a mixture with CO2 concentrations > 50 vol % (Procesi et al., 2019), and it may have the features of a hydrothermal system, with large amounts of water vapour (as in the case of the Lusi system in Indonesia; Mazzini et al., 2012).
- b.
Artesian systems. At mud spring sites, overpressured water displaces fine-grained sediments during upwelling, forming localised pools. These are generally shallow systems (tens to a few hundred metres deep), with surface manifestations within the range of some metres and mud flows whose extension may vary depending on the terrain morphology. These springs do not necessarily contain gas (e.g. Bristow et al., 2000). Dewatering structures like sand volcanoes may also display conical shaped features reaching > 10 m in size. These are shallow-rooted structures resulting from the remobilisation of shallow non-consolidated sediments (Gill and Kuenen, 1957).
- c.
Sedimentary diapirism. Here shales are purely driven by gravitative instability (similar to salt diapirism), without the need for gas overpressure; the movement is continuous but extremely slow and does not produce fluid eruptions or episodic activity on the surface (e.g. Bouriak et al., 2000; Bulanova et al., 2018).
- d.
Injected sands are rapid phenomena mostly triggered by sand fluidisation due to earthquakes, do not require gas overpressure, and do not show the presence of mud breccia. They may also be called sand injectites, they occur at depth and require the fracturing of overburden sediments through which fluidised sands migrate, eventually reaching the surface (i.e. extrudites; Jolly and Lonergan, 2002; Hurst et al., 2006; Polteau et al., 2008).
- e.
Pingos are morphological deformations of the ground, typically associated with frozen aquifers (so they exclusively exist on Earth in high-latitude regions) and with the presence of permafrost or gas hydrates; they are quite shallow and do not imply relevant transport of sediments but rather only surficial deformation. Their morphology may resemble that of small mud volcanoes. Although gas is not necessary to form a pingo (which is essentially a hydrological phenomenon), methane can be released when pingos are emplaced along faults or at pockmarks sites (Andreassen et al., 2017; Hodson et al., 2020).
2.3 Morphologies, rheology, and mud flows in sedimentary volcanism
Mazzini and Etiope (2017) provide an overview of the dimensions (from the metre scale up to 12 km in diameter) and morphologies observed at numerous mud volcano sites worldwide and propose a classification identifying the main processes that control the ultimate shape of the structures and the extensions of the mud flows. These processes are directly connected with the mechanisms of eruption/erosion that characterise each site and can be applied also to the other surface mud-fluid manifestations described herein. Dynamic factors include the eruption frequency and vigour (i.e. more explosive events vs less destructive events). The amount of gas/water/sediment rocks released during the eruptions will affect the rheology of the mud and, accordingly, the ultimate morphology of the flows. The local pre-existing topography of the eruptions will affect the shape of the surface manifestations. Mechanical factors include (a) the interaction between the erupted media (more or less viscous) and the surface (more or less erodible) hosting the flows, (b) the type of erosion (e.g. caused by submarine currents, wind, or rain) that is different depending on the geographical setting, or (c) the type of subsidence associated with subsurface dynamics. Different albedos observed at the mud flows can be used to infer the erosive efficiency of the erupted media (e.g. Mazzini et al., 2021) or the chronology of the eruptive phases (Mazzini et al., 2009).
2.4 Answering the key questions
With the clarifications given above, and based on numerous observations and studies on mud volcanoes worldwide, we will now try to answer two key questions about sedimentary volcanism.
- a.
Does surface mud always stem from a deep shale mobilised (diapir) along a fault?
- b.
Is the presence of gas necessary?
(a) On Earth, at least for relatively large (tens to thousands of metres in diameter) structures, it has been documented that the main mud component typically originates from deep shale units, upwelling even from depths of several kilometres, as demonstrated by studies conducted on mud breccia deposits (e.g. Cita et al., 1981; Inan et al., 1997, Akhmanov et al., 2003). Mud breccia and faults appear to be essential components of sedimentary volcanism. For small structures, a few centimetres or metres wide, the mud can be shallower and the process may be more similar to an artesian system, described above. Accordingly, the answer would be yes for traditional and relatively large sedimentary volcanoes, with mud breccia containing clasts and biomarkers transported from great depths.
(b) All non-ambiguous (and relatively large) mud volcanoes on Earth systematically release methane (and other gaseous hydrocarbons), because they develop within petroleum systems. However, as mentioned above, some manifestations may also release substantial amounts of CO2 (hybrid SHGS) or N2 (uplifted basins). Therefore, the answer to the second question is also yes. On Earth, sedimentary volcanism is always associated with gas emissions, as the gas is fundamental for rapid and episodic shale mobilisation (Fig. 2; see also Mazzini and Etiope, 2017). Gas may not be necessary for those peculiar structures, such as sedimentary diapirism and injected sands or other dewatering structures, described above, whose genetic mechanisms are different. Fundamentally, they do not have the “eruptive and fluid discharge” character that is typical of sedimentary volcanism and therefore are not classified ad such.
In conclusion, literature reviews from terrestrial mud volcanoes (e.g. Mazzini and Etiope 2017) reveal the key importance of the size of the emission structure as well as the presence of gas, mud breccia, and faults as relevant factors in understanding the genetic process driving mud-fluid manifestations and ultimately in providing a correct classification. Larger (tens to hundreds of metres wide) structures imply deeper roots, and their formation needs relevant fluid pressures and mobilisations of large volumes of sediments. Smaller structures (less than a few metres wide) may represent shallower processes, possibly involving local aquifers and gas in the shallow subsoil. Since many of the above parameters cannot yet be directly measured or observed on Mars, the use of the term “mud volcanism” remains unresolved and needs to account for significant potential unknowns since it may not completely satisfy the “terrestrial” definition. For these reasons, the broader term “mud-volcano-like structures” is the terminology recommended by the authors of this paper. The “size”, “mud breccia”, and “fault” factors, observable in available images from Mars, shall be carefully considered in the interpretation of the mud-volcano-like structures on the Red Planet, as discussed below. The release of gas can only be detected by on-site ground measurements (rovers) or, in the case of substantial gas plumes, by orbiters (Oehler and Etiope, 2021).
In this section, we briefly summarise the current knowledge about the morphology of mud-volcano-like structures on Mars (Table 1). Four individual subsections focus on (a) sub-kilometre- to kilometre-scale circular mounds widely spread across the northern lowlands, (b) kilometre-scale topographically positive features of various shapes often associated with flow-like features, (c) kilometre-scale flows (KSFs), and (d) hundreds of kilometre-long flows and deposits. These divisions were made in order to group features that bear similar morphological, morphometrical, and spatial similarities, although overlapping characteristics in some parameters often exist among these groups. Additionally, it should also be noted that edifices when compared among each other within individual fields as well as among different fields often show transition in their shapes. Hence, there is often no strictly bound variance among them and members of different groups can be present in one particular field.
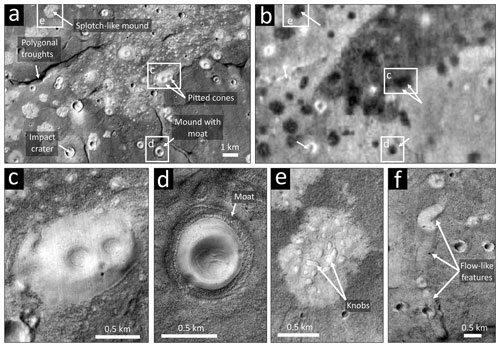
Figure 3Sub-kilometre- to kilometre-scale circular mounds in Acidalia Planitia. (a) A variety of types of bright mounds. Centred 41.12∘ N, 26.34∘ W. Rectangles show locations of (c–e). (b) Same area as (a), showing dark responses of the bright mounds and surrounding materials in nighttime IR. Arrows as in (a). Compare Fig. 3a and b to see the different responses in nighttime IR of mounds and impact craters. (c) Pitted cones showing central depressions and material on flanks apparently superposed on the darker substrate of the plains. (d) High Resolution Imaging Science Experiment (HiRISE) image (ESP_026732_2215_RED) showing a bright mound with a central depression and surrounding moat. (e) Bright splotch-like mound with entrained, boulder-sized angular knobs. (f) MRO Context Camera (CTX) image (J03_045945_2201_XN_40N027W) showing flow-like features extending from a bright mound (top arrow) to darker apparent flows (lower two arrows). Centred 40.25∘ N, 27.13∘ W; location ∼ 62 km SSW of (a). North is up in all panels. Panels (a), (c), and (e) are from CTX mosaics (NASA/JPL/Malin Space Science Systems; © Google Earth/Mars). Panel (b) is from the Mars Odyssey Thermal Emission Imaging System (THEMIS) nighttime infrared (IR) global mosaic, v. 14 (NASA/JPL-Caltech/Arizona State University). Panel (d) is an MRO HiRISE image (NASA/JPL/University of Arizona). Panel (f) is an MRO CTX image.
3.1 Morphologic expression of putative sedimentary volcanism on Mars
3.1.1 Sub-kilometre- to kilometre-scale circular mounds
Many tens of thousands of bright, sub-kilometre- to kilometre-scale circular mounds occur in the northern plains of Mars (Fig. 3). The largest abundances are in Acidalia and Utopia planitiae, but additional examples occur in Chryse and Arcadia planitiae, Cydonia Mensae, the Isidis–Utopia overlap, Isidis Planitia, and possibly in the Scandia region (e.g. Scott and Tanaka, 1986; Davis and Tanaka, 1995; Tanaka, 1997, 2005; Tanaka et al., 2000, 2003, 2008; Farrand et al., 2005; Kite et al., 2007; Rodríguez et al., 2007; Skinner and Tanaka, 2007; Allen et al., 2009, 2013; McGowan, 2009; Oehler and Allen, 2009, 2010, 2011, 2012a, b; Skinner and Mazzini, 2009; Komatsu, 2010; McGowan and McGill, 2010; Allen et al., 2013; Ivanov et al., 2014; Orgel et al., 2015, 2019; Komatsu et al., 2011, 2016; Hemmi and Miyamoto, 2018; De Toffoli et al., 2019, 2021). While the great majority of these mounds occur in the northern lowlands, possible examples have been reported for several localities in the highlands, including craters in Arabia Terra (Pondrelli et al., 2011; Franchi et al., 2014) and a flat-floored depression in Terra Sirenum (Hemmi and Miyamoto, 2017).
The mounds have typically been compared to a variety of terrestrial analogues (e.g. rootless cones, scoria cones, tuff cones, pingos, erosional remnants, clathrate degasification structures, and mud volcanoes), and in many cases, a mud volcano interpretation has been deemed most consistent with the observed morphologies, geologic setting, associated flow structures, and evidence of a fine-grained (low thermal inertia) sediment size.
The mounds in Acidalia Planitia (Fig. 3) were described in detail by Oehler and Allen (2010), using the Thermal Emission Imaging System (THEMIS) nighttime IR dataset, which has a 100 m per pixel resolution, allowing identification of features with diameters of greater than ∼ 300 m. Checks were made with higher-resolution datasets where possible (e.g. Mars Orbital Camera, MOC; THEMIS Visible, VIS; MRO Context Camera, CTX, resolution 5–6 m per pixel; and the High Resolution Imaging Science Experiment, HiRISE, resolution ∼ 30 cm per pixel). In that study, the total area of their occurrence was outlined, and in about half of that area, more than 18 000 examples were mapped. Based on that, ∼ 40 000 such features were estimated for southern Acidalia Planitia. Hemmi and Miyamoto (2018) studied 1300 mounds in southern Acidalia, using 40 HiRISE-based digital elevation models (DEMs) to measure mound heights and basal diameters. The HiRISE data allow identification of features > ∼ 1 m in diameter. These two studies provide the following observations: the mounds in Acidalia (Fig. 3) are circular to subcircular in plan view, with diameters ranging from ∼ 0.3 to 2.2 km (average ∼ 0.8 km). The heights of the examples measured by Hemmi and Miyamoto (2018) average 15.2 m, with a range of 1.1 to 69.5 m. In a cross-sectional profile, many (at least about half of those observed) of the mounds appear as domes (Fig. 3a), commonly displaying a central depression (Fig. 3c and d), or steep-sided cones. In some areas, the mounds are surrounded by moats (Fig. 3d). Some bright circular mounds may appear to be nearly flat and more irregular in plan view, and these can sometimes enclose large boulder-like knobs (Fig. 3e). These types of mounds were described as early as 1986 by Scott and Tanaka and in 1991 by Scott and Underwood. Farrand et al. (2005) described these morphologies as domes, cones, and splotches. Most have a high albedo and smooth surfaces relative to the surrounding plains. Some have apron-like extensions of the smooth, high albedo material onto the plains. Similar smooth-textured, high-albedo material forms occasional lobate flow-like structures that emanate from some of the mounds (Fig. 3c and f). THEMIS nighttime infrared (IR) images show the mounds to be dark in nighttime IR (compare Fig. 3a and b), implying that their thermal inertia is lower than that of the plains and likely reflects a finer grain size than that of the plains (Oehler and Allen, 2010). Farrand et al. (2005) concluded that “dried, loosely cemented, mud deposits would be a good match to both the albedo and thermal inertia of these mounds”; their work was based on MOC Narrow Angle (1.5 to 6 m per pixel), THEMIS VIS (19 m per pixel) images, and a Thermal Emission Spectrometer (TES)-derived thermal inertia map (20 pixels per degree). The mineralogy of the bright mounds in Acidalia has been investigated with data from the Compact Reconnaissance Imaging Spectrometer for Mars (CRISM), but results have not been definitive, possibly due to enhanced coatings of poorly crystalline materials (Oehler and Allen, 2010).
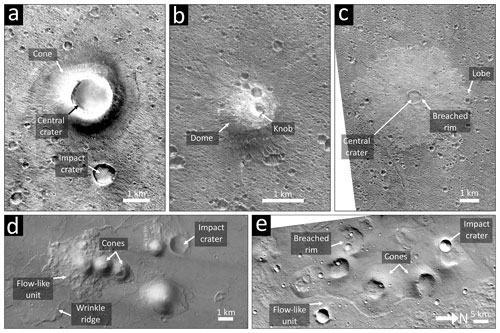
Figure 4Examples of kilometre-scale features from various regions of Mars. Panel (a) shows a conical feature with steep flanks and a wide central crater (HiRISE ESP_022025_2000, centred 19.73∘ N, 322.44∘ E), (b) a domical feature with a small central knob in the summit area (HiRISE ESP_025137_1995, centred 19.04∘ N, 322.64∘ E), (c) a shield-like or pie-like feature with a central breached crater (HiRISE ESP_025704_2005, centred 20.186∘ N, 321.259∘ E), (d) a small cluster of conical and domical edifices associated with a flow-like unit, and (e) a cluster of wide conical edifices with wide breached central craters surrounded by a flow-like unit. Edifices on (a–c) are situated in the southern part of Chryse Planitia. The fields of cones in panels (d) and (e) are situated on the floor of Coprates Chasma (CTX P13_006269_1670_XN_13S062W, centred 12.711∘ S, 297.67∘ E) and in the Amenthes/Nepenthes region (CTX G01_018499_1961_XN_16N252W, centred 16.194∘ N, 107.373∘ E) respectively. Except for panel (e), north is up. HiRISE imagery: NASA/JPL/University of Arizona and CTX imagery NASA/JPL/Malin Space Science Systems.
3.1.2 Cones, domes, and shields associated with flow-like units
Members of this group have been reported in Valles Marineris in Candor and Coprates chasmata (Harrison and Chapman, 2008; Chan et al., 2010; Okubo, 2016; Kumar et al., 2019; Wheatley et al., 2019), in the southern part of Chryse Planitia (Komatsu, 2010; Komatsu et al., 2011, 2016; Brož et al., 2019), in the Amenthes/Nepenthes region (Skinner and Tanaka, 2007), and in Hydraotes Chaos (Meresse et al., 2008). Edifices in these regions display a wide range of shapes (Fig. 4), both among individual fields and when compared between them. Some edifices have a conical morphology including well-developed summit craters (Fig. 4a), while others have no such craters (Harrison and Chapman, 2008; Chan et al., 2010; Okubo, 2016; Kumar et al., 2019; Wheatley et al., 2019). Still other structures have the cross-sectional shape of domes with steep sides and flat summit areas that may feature small central knobs (Fig. 4b). Additional morphologies include shield- or pie-like edifices with one or multiple summit craters and well-developed lobate margins (Fig. 4c; Komatsu et al., 2016; Brož et al., 2019). The central craters associated with kilometre-scale cones and shield-like edifices might be either enclosed (Fig. 4a) or breached (Fig. 4e). In several regions, some of these edifices are associated with flow-like units over which they might be superposed (Fig. 4d; Okubo, 2016; Brož et al., 2017) or be embayed by (Fig. 4e; Skinner and Tanaka, 2007; Brož and Hauber, 2013). These flow-like units are well developed, contain lobes, and are within the range of a few hundred up to a few kilometres in size. Edifices of this group also have broad variations in their sizes, both among features in individual fields as well as among various fields. For example, the field of ∼ 130 conical features mapped based on CTX mosaic within Coprates Chasma range in size from 0.2 to 2 km in diameter, with average of 0.8 km (based on CTX data measurements of 59 edifices; Brož et al., 2017). Edifices are from 75 to 250 m high and their flanks are between 15 and 25∘ (based on HiRISE DEM investigation of six edifices; Brož et al., 2015a). On the other hand, the field of ∼ 170 pitted cones in the Amenthes/Nepenthes region is composed of edifices that are much wider than those in Coprates Chasma. They range from 3 to 15 km in diameter (average 7.8 km, based on measurements of 92 edifices by utilising CTX and High Resolution Stereo Camera (HRSC) images; Brož and Hauber, 2013). Based on HRSC DEMs, they are also higher, from 30 to 370 m (average ∼ 120 m, based on measurements of 53 edifices) and their slopes are typically below 10∘. Features among this field also have much wider craters as compared to those in Coprates Chasma, and the craters are often breached. The crater floors can be at or a few dozen metres below the preeruptive level of the surrounding plains (observed for 13 edifices out of 47 measured; for details, see Table S.1 in the auxiliary material in Brož and Hauber, 2013). More than 1300 + structures with sizes ranging from 0.2 up to 20 km have been mapped based on the CTX data in the southern part of Chryse Planitia. They have been classified into five different types – cratered cones, shield-like edifices, domes with a small central knob on their summit craters, irregular pies without significant topographical expression, and KSFs (Brož et al., 2019). However, in some cases, the edifices show transitional morphologies between these defined classes.
In this group, edifices do form fields extending commonly over hundreds of kilometres, but the number of individual edifices in these fields is relatively small and hence their spatial density is low. For example, the field in the southern part of Chryse Planitia composed of 1300 + structures is spread over 700 000 km2 (Brož et al., 2019), giving a spatial density around ∼ 0.02 structure per 1000 km2 only. Similarly, the western part of the field in Coprates Chasma is composed of 124 edifices and spread over an area of about 155 km × 35 km (Brož et al., 2017), hence ∼ 5400 km2, so the spatial density is around ∼ 0.2 structures per 1000 km2. On the other hand, kilometre-scale circular mounds described in Terra Sirenum and in Acidalia Planitia show the spatial density ∼ 2.1 structures per 1000 km2 (Hemmi and Miyamoto, 2017) and between ∼ 0.2 and ∼ 1.1 structures per 1000 km2 (Oehler and Allen, 2010) respectively. So their spatial density is generally lower than for cones described in previous group (see Table 1 for more details).
Finally, features in this group occur in a variety of geologic settings, inside the network of interconnected canyons of Valles Marineris (Harrison and Chapman, 2008; Okubo, 2016), large-scale chaos terrains (Meresse et al., 2008), at the terminations of large outflow channels (Komatsu et al., 2016; Brož et al., 2019), or on circumferential annular spaces of large impact basins (Skinner and Tanaka, 2007). These geological settings are generally quite different from the huge basins in Acidalia and Utopia that accumulated thick, distal facies sediment piles from the outflow floods.
The differences in geologic settings, morphology and spatial density may argue that this group of features may have a different origin than the previous group. Because they have a similar variety of shapes and are commonly associated with flows, it is common for terrestrial kilometre-scale mud volcanoes to be proposed as potential analogues (e.g. Jakubov et al., 1971; Skinner and Tanaka, 2007; Okubo, 2016; Aliyev et al., 2015; Komatsu, 2010; Komatsu et al., 2011, 2016; Mazzini and Etiope, 2017; Brož et al., 2019; Kumar et al., 2019; Wheatley et al., 2019). However, igneous volcanism has been invoked as an alternative mechanism for their formation in several cases (Lucchitta, 1990; Meresse et al., 2008; Brož and Hauber, 2013; Brož et al., 2015a, b, 2017).
The spectral data from CRISM have been used to gain insight into their formation mechanism; however, to date, spectral observations do not support a sedimentary origin over an igneous one, or vice versa. This is because data do not unambiguously show the presence of phyllosilicates, carbonates, or sulfates in association with these edifices (Dapremont and Wray, 2021; see also Brož et al., 2017, for additional discussion).
3.1.3 Kilometre-scale flows
KSFs have been identified on several martian low plains: Chryse (Komatsu et al., 2011, 2016; Brož et al., 2019, 2022a), Acidalia (Ivanov et al., 2015; Ivanov and Hiesinger, 2020), Utopia (Ivanov et al., 2014, 2015), and Elysium planitiae (Wilson and Mouginis-Mark, 2014) and at the highland–lowland boundary (HLB) between southern Utopia Planitia and Terra Cimmeria (Skinner and Tanaka, 2007; Skinner and Mazzini, 2009). They represent a morphologically diverse group of landforms characterised by (a) spatially extensive spreading controlled by local topography and (b) the presence of lobate margins indicative of flow processes. In some martian volcanic provinces, like Tharsis or Elysium, such landforms have been interpreted as lava flows (e.g. Hartmann and Berman, 2000; Bleacher et al., 2007; Keszthelyi et al., 2008; Hauber et al., 2009, 2011). Based on interpretation of remote sensing data, however, subsurface sediment mobilisation has been proposed as the plausible formation mechanism for some of these features as well (e.g. Skinner and Tanaka, 2007; Ivanov et al., 2014, 2015; Wilson and Mouginis-Mark, 2014; Komatsu et al., 2011, 2016; Okubo, 2016; Brož et al., 2019).
KSFs are often elongated in plan map view. This elongation is interpreted to be the result of material released from a source area and flowing down the local topographic gradient and filling nearby terrain depression(s). However, in flat areas of Chryse and Utopia planitiae, their length-to-width ratio is lower compared to KSFs found on inclined surfaces. KSFs vary greatly in areal extent and may feature individual or overlapping flow-like structures (e.g, Komatsu et al., 2016; Brož et al., 2022a). The largest examples are situated within Utopia Planitia. Here, many overlapping flows spread over areas ranging from 100 to 1000 km2 (Cuřín et al., 2023). However, similar extents have been found for individual KSFs such as Zephyria Fluctus, which has an area of 153 km2 and an average thickness of 3.8 m (based on a combination of Mars Orbiter Laser Altimeter (MOLA) data and a DEM constructed from stereo pairs of CTX images; Wilson and Mouginis-Mark, 2014). This unique flow has been interpreted to be formed by the emplacement of mud by Wilson and Mouginis-Mark (2014). Individual flows in Utopia Planitia typically cover an area of ∼ 35 km2 and have cross-sectional shapes of plateaus standing above surrounding terrains to heights of 20–30 m (based on CTX DEMs; Cuřín et al., 2023). These are comparable to the examples found in Acidalia Planitia and Utopia–Cimmeria HLB (Skinner and Tanaka, 2007; Skinner and Mazzini, 2009). The flows present in Chryse Planitia are smaller (few tens of square kilometres; Brož et al., 2019).
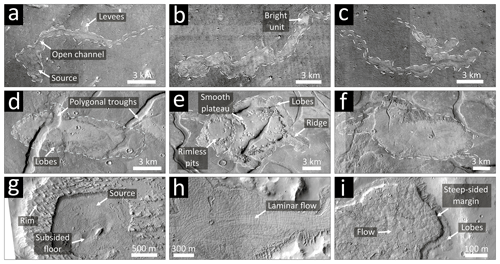
Figure 5An example of kilometre-sized flows (KSFs) from three regions of Mars showing large variability in their shapes and general appearance. While the KSFs within Chryse Planitia captured in (a–c) consist of three different parts – source area, open channel, and levees – and are often associated with bright smooth units, the KSFs in Adamas Labyrinthus (d–f) do not show such an appearance. Instead they have the shape of a plateau. Panels (d–f) show plateau-shaped KSFs from Adamas Labyrinthus that are standing above the surrounding terrain to a height of 20–30 m and they do not show clear source area from which the material originated. A unique KSF is the flow of Zephyria Fluctus, which shows a well-developed large source area (g), a former flow pattern on its surface (h), and well-developed steep-sided margins and lobes (i). Panel (a) is based on CTX image P17_007639_1997_XN_19N034W (centred 19.85∘ N, 326.02∘ E), (b) CTX F01_036121_2011_XN_21N034W (centred 20.41∘ N, 325.69∘ E), (c) CTX B19_016856_1990_XI_19N035W (centred 20.228∘ N, 324.05∘ E), (d) CTX G21_026424_2175_XN_37N257W (centred 102.2∘ E, 37.52∘ N, 102.2∘ E), (e) CTX P17_007779_2181_XN_38N259W (centred 39.14∘ N, 100.92∘ E), (f) CTX P17_007502_2195_XI_39N256W (centred 38.63∘ N, 104.2∘ E), (g) HiRISE ESP_037169_1805 (centred 0.59∘ N, 155.29∘ E), (h) HiRISE ESP_027464_1805 (centred 0.65∘ N, 155.39∘ E), and (i) HiRISE ESP_028941_1810 (centred 0.795∘ N, 155.592∘ E) respectively. HiRISE imagery: NASA/JPL/University of Arizona and CTX imagery NASA/JPL/Malin Space Science Systems.
Most KSFs comprise central and marginal units, each with specific morphologies depending on the specific region on Mars. In Utopia and Acidalia, KSFs are characterised by smooth central flat parts with randomly distributed rimless depressions (Fig. 5e; Ivanov et al., 2015) and marginal units consisting of coalescing pits, distributary branching, and lobate hummocks (Fig. 5e and f). The KSFs of the Utopia–Cimmeria HLB consist of lobate material with varying thickness (Skinner and Mazzini, 2009), but the difference between their central and marginal units is not as pronounced. Zephyria Fluctus' central laminar flow unit has a unique polygonal surface texture with steep-sided margins (Fig. 5h and i; Wilson and Mouginis-Mark, 2014). The channelised flow-like features of Chryse Planitia are more complex and consist of three morphological elements: a central depression, leveed channels, and a distal portion of the fading channel(s) (Fig. 5a; Komatsu et al., 2016; Brož et al., 2019; 2022a). Topographically low mounds inside the central depressions have been interpreted to mark the positions of feeder vents (Brož et al., 2022a). Similarly, Zephyria Fluctus seems to be sourced from a circular pond-like depression (Fig. 5, Wilson and Mouginis-Mark, 2014).
In Acidalia and Chryse planitiae, KSFs are often associated with circular mounds. A similar spatial association is observed in Utopia Planitia, where plateau-like outflows are often emanating from elongated ridges (Cuřín et al., 2023). In Utopia and Acidalia planitiae, KSFs appear in the proximity of craters with pancake-like ejecta, ghost craters (Ivanov et al., 2015), and giant polygons (Ivanov et al., 2014; Cuřín et al., 2023).
KSFs are discernible in THEMIS nighttime IR imagery. Across all mentioned locations, the central units are darker than the marginal ones, but their contrast to the surrounding terrain varies. In Chryse and Utopia planitiae, they appear darker than the surroundings, while KSFs in Acidalia Planitia as well as Zephyria Fluctus appear brighter than the surrounding landforms. Using THEMIS nighttime IR data, Komatsu et al. (2011) examined the material forming one of the KSFs within Chryse Planitia and the associated conical and dome-shaped edifices. They found that these features had a lower thermal inertia (i.e. potentially finer grain size) than the surrounding units, which they interpreted as indicative of a relatively finer grain size.
3.2 Distribution and geologic setting
Martian mud-volcano-like features have mainly been observed in various parts of the northern lowlands of Mars, especially in Utopia, Chryse, and Acidalia planitiae. For example, in Acidalia, where extensive mapping of more than 18 000 mounds has been completed (Oehler and Allen, 2012a), the circular mounds commonly occur at elevations below −4000 m in locations associated with giant polygons, which also are almost exclusively found in the northern plains of Mars (Oehler and Allen, 2012a; Allen et al., 2013; Moscardelli et al., 2012). The mounds occur individually, in pairs, in irregular clusters, and in chains. The chains may be relatively rectilinear in plan view, overlying troughs forming giant polygons (Gallagher et al., 2018), or they may be curvilinear, aligned along arcuate ridges in a type of landform termed “thumbprint terrain” (Guest et al., 1977). Facies analysis incorporating catchment areas and transport distances of sediment deposition from the Noachian to Hesperian fluvial systems and Late Hesperian outflow floods predicts that southern Acidalia would be the depocentre for fine-grained, distal facies muds (Rice and Edgett, 1997; Allen et al., 2013; Oehler and Allen, 2010, 2012a, b; Oehler et al., 2021). The long transport distances from Noachian catchments in the highlands to depocentres in southern Acidalia would promote excellent grain size separation such that distal facies sediments in southern Acidalia would be expected to contain thick accumulations of mud. This prediction is supported by Salvatore and Christensen (2014a, b), who used high-resolution datasets to investigate morphology and spectral signatures and concluded that southern Acidalia is a region of extensive, fine-grained, and water-saturated sedimentation, and Ivanov and Hiesinger (2020), who conducted a photogeological study along with crater size–frequency distributions and concluded that a volatile-saturated, mud-rich unit was deposited in the southern Acidalia plains. Thumbprint terrain in two regions of Acidalia has been interpreted as the result of impact-generated tsunamis (Rodríguez, et al., 2016; Costard et al., 2017), supporting the concept of a northern ocean and water-saturated sediments in the Acidalia depocentres. The mounds that occur in curvilinear chains in this terrain have been interpreted as mud-volcano-like structures formed by rapid compaction in high-energy flows caused by tsunami-producing impacts (Di Pietro et al., 2021).
After deposition of Noachian to Hesperian fluvial units within and below (a) the region now covered by the Vastitas Borealis Interior and Marginal units of Tanaka et al., 2005 or (b) the Late Hesperian lowlands unit (lHl) of Tanaka et al., 2014, the Late Hesperian, circum-Chryse outflow floods would have injected enormous quantities of sediments into the same area (Lucchitta et al., 1986; Baker et al., 2015; Alemanno et al., 2018). Both the earliest outflow sediments and the older underlying strata would have been rapidly buried. On Earth, rapid burial of a volatile- and mud-rich section is ideal for development of subsurface overpressure and initiation of MV (Kopf, 2002). The circum-Chryse outflow sediments could have done the same on Mars (Oehler and Allen, 2010). This process might additionally explain the approximate co-location of bright mounds and giant polygons in Acidalia (Oehler and Allen, 2012a; Allen et al., 2013; Orgel et al., 2019).
The Chryse outflow floods and sediment emplacement might also be responsible for the formation of kilometre-scale cones, domes, and shields situated in the southern part of Chryse Planitia, in a region near the termini of several large outflow channels, namely in the Simud, Ares, and Tiu valles (Komatsu et al., 2011, 2016; Pajola et al., 2016). Here, more than 1300 + edifices, classified into five different types (including kilometre-sized cones, domes, shields, and KSF), spread over the area of 700 000 km2 (Brož et al., 2019). The distribution of these features shows that they are clustered and anticorrelated to the erosional remnants of ancient highlands, suggesting a genetic link between their distribution and the sedimentary deposits over which they are superposed. Their distribution also shows that different types of features occur preferentially at specific latitudes (Fig. 5 in Brož et al., 2019), although the area of their extension often overlaps with regions populated also by other types. Such distribution patterns could be related to a model of sandar facies as suggested for this region by Rice and Edgett (1997). These authors identified three facies types (proximal, midfan, and distal facies) in a lateral sequence progressing from south to north. Most of the known features were mapped in the zone of the midfan facies; however, no clear correlation between feature type and distance to outflow channel termini was found (Brož et al., 2019).
Another distinct distribution pattern was found for a field of ∼ 170 pitted cones and 80 + smaller mounds (Skinner and Tanaka, 2007; Brož and Hauber, 2013) in the Amenthes/Nepenthes region situated close to the dichotomy boundary, between the cratered highlands of Tyrrhena Terra in the south and smoother plains of Utopia Planitia in the north. Here, the edifices are aligned, from west to east, in the NW–SE and then W–E direction parallel to the southern margin of Utopia Planitia. To explain such a distribution, Skinner and Tanaka (2007) proposed the existence of annular ring basins in an impact tectonics scenario that would have acted as locations for sediment accumulation in southern Utopia Planitia and hence a source reservoir for sedimentary volcanism. In addition, the field of ∼ 130 pitted cones reported in Valles Marineris in Coprates Chasma (Harrison and Chapman, 2008; Chan et al., 2010; Okubo, 2016; Brož et al., 2017; Kumar et al., 2019; Wheatley et al., 2019) shows aligned distribution in the NW–SE direction. Brož et al. (2017) proposed that such distribution is controlled by structures oriented roughly parallel to the long axis of the Coprates Chasma tectonic graben.
The south-western part of Utopia Planitia, in the region of Adamas Labyrinthus, also displays evidence of possible sedimentary volcanism in the form of a field of more than 300 of KSFs. They were firstly described by Ivanov et al. (2014), who referred to them as “etched flows”. An additional mapping campaign performed by Cuřín et al. (2023) categorised these KSFs into four classes (“hills”, “ridges”, “plateaus”, and “complexly layered units”). Several KSFs can also be found throughout Acidalia Planitia southward of Acidalia Mensae (Ivanov et al., 2015; Ivanov and Hiesinger 2020), as well as around the Utopia–Cimmeria HLB (Skinner and Tanaka, 2007; Skinner and Mazzini, 2009), although no comprehensive inventory of their presence in these regions exists. In Elysium Planitia, the single flow of Zephyria Fluctus with a supposed sedimentary origin is present within the lower unit of the Medusae Fossae Formation (Fig. 5g–i; Wilson and Mouginis-Mark, 2014).
It remains unclear at which depth(s) the source reservoir(s) for the hypothesised sedimentary volcanoes is/are located. Hemmi and Miyamoto (2018) estimated source depths for the mounds in Acidalia to be 110–850 m (if the mounds were formed subaerially) and 30–450 m (if the mounds were formed sub-aqueously). Their work was based on bulk densities, fractures associated with co-located giant polygons, and an isostatic compensation model where the depth of the mud source was estimated from mound heights. De Toffoli et al. (2019) estimated source depths for mounds in Arcadia Planitia of 16–18 km. Their work was based on fractal analysis of the mounds to assess whether their spatial distributions were consistent with control by underlying fractures and then on the assumption that upper cut offs determined by the fractal analysis reflect the depths of the fluid source. The orders-of-magnitude difference in the estimated depths to the source reservoirs of these two studies highlights uncertainties in both the approaches utilised for these assessments as well as in the understanding of the origin of the bright mounds in the northern plains of Mars.
3.3 Ages
With the exception of KSFs, the age of martian mud-volcano-like structures is difficult to determine as they do not represent units of sufficient size for crater counting (e.g. Warner et al., 2015). Moreover, many of them have a relatively rugged topography with steep slopes. Hence, it is typically only possible to date spatially larger units with a known relative stratigraphic position with respect to the hypothesised mud volcanoes (i.e. either the edifices are superposed on these units or are partly buried/embayed by them; e.g. Brož and Hauber, 2013; Brož et al., 2019). This approach enables bracketing their ages by maximum and minimum ages; however, this approach is commonly fraught with large uncertainties.
This can be illustrated by the example of circular mounds in Acidalia. In most areas, these mounds have erupted onto the Vastitas Borealis Formation (VBF), a Late Hesperian to Early Amazonian (∼ 3.2 to 1.75 Ga) unit interpreted as either a palaeo-ocean deposit (Kreslavsky and Head, 2002) or a mixture of Noachian to Hesperian materials and local outflow channel sediments (Tanaka et al., 2003). Since the majority of the mounds overlie the VBF, they must be younger than its Late Hesperian to Early Amazonian age. Nevertheless, the minimum age has not been established. Some studies interpret the mounds as generally ancient features that formed on early Mars while fluids were still abundant in the shallow subsurface of the northern plains and perhaps while an ocean existed (e.g. Oehler and Allen, 2010, 2012a; Allen et al., 2013). This interpretation would be consistent with the interpretations of thumbprint terrain as a product of tsunamis. However, this view has been challenged by Rodríguez et al. (2019), who suggest a later stage of sedimentary volcanism that postdates a possible ocean. Supporting a more recent origin of the features, De Toffoli et al. (2019) proposed that relatively small mounds (0.3 to 0.5 km in diameter) associated with thumbprint terrain in Arcadia Planitia may be young, with a “last occurrence” of ∼ 370 Ma. That age is based on craters < 1 km in diameter, which because of their small size, could reflect a resurfacing age rather than a formation age (see Warner et al., 2015, for discussion of crater sizes and age interpretations).
Similar uncertainties are associated with the ages of kilometre-sized cones, domes, shields, and KSFs. Specifically, the features within the southern part of Chryse Planitia are spread over a unit that significantly resurfaced in 3.2 Ga (Brož et al., 2019) and that experienced at least another two resurfacing events. As the features seem to have formed after these resurfacing events and they are partly covered by secondaries formed during the formation of the Mojave crater, which has been dated to 4.7 Ma (Werner et al., 2014), this dates them to a range of between 880 and 5 Ma (Brož et al., 2019). Similarly, cones within Coprates Chasma were emplaced on top of sedimentary deposits of Hesperian age (Okubo, 2016), but again the unit later resurfaced and the cones formed after this resurfacing event. So they are likely Middle to Late Amazonian in age since some are superposed by a young landslide (Brož et al., 2017). However, some known edifices might be older, like pitted cones in the Amenthes/Nepenthes region that date before ∼ 2.4 Ga (Brož and Hauber, 2013).
The ages of KSFs in Utopia and Acidalia are similarly uncertain. Ivanov et al. (2015) tied their formation to the later stages of VBF emplacement; hence, they should be from 3.57 and 3.61 Ga respectively. Zephyria Fluctus, situated within the Late Hesperian transition unit (Tanaka et al., 2005, 2014), has the visual appearance of a very recent flow (Mouginis-Mark, 2013), but its precise age has not yet been determined. However, it should be noted that remote sensing methods based on absolute or relative techniques are still not entirely accurate tools for determining age, especially for small-scale edifices. Readers should therefore approach the above data with caution.
3.4 Effect of the environment on sedimentary volcanism
Mars is a planet with very different environmental properties as compared to Earth. Both the surface gravitational acceleration and the current atmospheric pressure are lower, at 3.71 versus 9.81 m s−2 and ∼ 600–1000 versus ∼ 105 Pa respectively. Today, the range of atmospheric pressure at the surface of Mars can reach likely up to 12.4 mbar at the deepest point on the bottom of the ancient impact basin, Hellas (Haberle et al., 2001; Wray, 2021) and can drop to 0.7 mbar on the top of the highest mountain, Olympus Mons. The surface temperature is on average −60 ∘C, but it can range from −143 ∘C at the poles up to +35 ∘C in equatorial regions. Although average temperatures are far below the freezing point of water, locally higher temperatures at favourable seasons might be reached, theoretically enabling liquid water to be present on the surface today (Wallace and Sagan, 1979; Brass, 1980; Carr, 1983; Haberle et al., 2001; Hecht, 2002; Möhlmann, 2004; Kossacki et al., 2006; Bargery et al., 2010). However, such water would likely be transient, because the low atmospheric pressure would trigger boiling, freezing, and eventually evaporation into the atmosphere (e.g. Hecht, 2002; Wray, 2021). These processes might have inhibited the ability of water to propagate over the martian surface during most of its history.
Initial studies of mud behaviour at martian surface pressure were performed by Wilson and Mouginis-Mark (2014). The authors proposed that the water present in the mud would be unstable and evaporate from the mud flow, ultimately removing the latent heat from the mixture. This implies that the residual water present in the mud mixture would freeze relatively quickly, in the range of hours to days. Additional insight came from the experimental work of Brož et al. (2020a, b), where the behaviour of low-viscosity mud was experimentally studied in a low-pressure chamber partly simulating the Mars environment. Their results showed that low-viscosity mud flows could actually propagate over cold (< 273 K) and warm (> 273 K) surfaces at martian atmospheric pressure, but the mechanism of such propagation would be different from that observed on Earth. On Mars, mud propagating over cold surfaces would rapidly freeze on the surface of the flow due to evaporative cooling (Bargery et al., 2010) forming an icy crust, leading to propagation in a similar manner to pahoehoe lava flows observed on Earth (Hon et al., 1994). Once such an icy crust has formed, the interior of the mud flow is protected from additional evaporative cooling. As a consequence, mud remains liquid inside the crust for prolonged periods of time and propagates via mud tubes (analogous to lava tubes; Calvari and Pinkterton, 1999). In contrast, low-viscosity mud propagating over a warm surface boils and levitates above the surface (Brož et al., 2020b). As the water content within martian mud flows might have varied, mud flows may have had different viscosities. Brož et al. (2022b) experimentally revealed that the exposure of high-viscosity mud to low atmospheric pressure also leads to the formation of an icy crust, but also to a volume increase. This phenomenon occurs since the low atmospheric pressure causes an instability of the water present in the mud mixture, leading to the formation of expanding bubbles, which cannot escape from the high-viscosity mud and increases the volume of the mud by up to 15 %. Hence, low-pressure experiments demonstrate that the propagation and behaviour of mud on Mars indeed differs distinctly from that on Earth. Sedimentary edifices built by mud flows on Mars are therefore expected to differ in shape and morphology from their terrestrial counterparts (Brož et al., 2019, 2020a, 2022a).
Useful insights to understand the mechanisms of mud flows at low atmospheric pressures might come from theoretical considerations of igneous volcanism on Mars, for which the roles of different gravity and atmospheric pressure have been intensively studied (e.g. Dehn and Sheridan, 1990; Wilson and Head, 1994; Wilson and Head, 2004; Parfitt and Wilson, 2008; Brož et al., 2021, and references therein). These investigations showed that a low surface pressure environment is capable of affecting the ascent of magma in the feeding conduit (e.g. Wilson and Head, 1994; Parfitt and Wilson, 2008). In fact, the low atmospheric pressure would favour the formation of bubbles and their growth within the ascending magma and hence reduce its density. Such a decrease would cause a larger density contrast to the surrounding rocks, hence in buoyancy, and would ultimately increase the speed of the magma's ascent. Similarly, rapid decompression of mud during its ascent together with rapid degassing might also favour its release via low-energy explosive eruptions. In this case, muddy eruptions similar in nature to Hawaiian and/or Strombolian igneous eruptions might be more frequent on Mars than on Earth because significant gas expansion within the final phase of its ascent might increase the ejection velocities of the expelled mixture (Wilson and Head, 1994).
As surface atmospheric pressure has varied on all timescales (for a recent review, see Jakosky, 2021) and temperatures were likely higher in the past (Haberle et al., 2017), martian mud flows might have been emplaced at times where the importance of evaporative cooling may have been significantly reduced. It is reasonable to expect that the shapes of martian sedimentary volcanoes may then vary significantly among various fields due to local environmental properties (Brož et al., 2022a; Cuřín et al., 2023). To our knowledge, there are no dedicated studies that have investigated such morphological variabilities. To perform such study is, however, particularly challenging since the ages of possible martian sedimentary volcanoes as well as the exact palaeo-pressures in Mars' history are only poorly constrained (e.g. Kite et al., 2014).
In addition to low atmospheric pressure, Mars also has a lower gravity as compared to Earth, and it remains unknown how this may affect sedimentary eruptions. Once again, a comparison to igneous volcanism can provide valuable hints. It has been shown that the lower gravity on Mars tends to reduce the speed of lava flows and increase their thickness, as the liquid would spread laterally to a lesser degree (Wilson and Head, 1994; Rowland et al., 2004). As a consequence, this would change the heat loss rate of the lava flow. In a similar manner, mud flows could form thicker accumulations without losing heat. Moreover, the low temperatures at the martian surface could also impede the ability of water to infiltrate into the subsurface (Conway et al., 2011; McCauley et al., 2002; Pfeffer and Humphrey, 1998), limiting water loss from the mud mixture and thus maintaining the viscosity of the flow (i.e. it does not become more viscous by losing water). Finally, the low atmospheric pressure would also reduce the importance of cooling the mud flows by convection of the overlying atmosphere.
The lower gravity on Mars could also hinder the occurrence of sedimentary volcanism, as the lithostatic pressure within the crust has a different gradient to that on Earth. In other words, in order to achieve the same pressure as at a certain depth on Earth, it is necessary to be approximately three times deeper on Mars (i.e. the sedimentary basins need to be three times thicker). Therefore, from the theoretical point of view, it might be more challenging to have suitable depths of strata for sedimentary volcanism to arise on Mars compared to on Earth.
There are also no analytical or numerical models to explain the process of sediment mobilisation on Mars, despite significant progress in analogue modelling over the last years. The main challenge is determining how the historical variations in the atmospheric surface pressure and the low gravity on Mars might affect this process. Namely, for mud-volcano-like edifices formed under atmospheric conditions inhibiting the presence of liquid water on the surface for a prolonged period of time, it is unknown how evaporative cooling and the subsequent formation of an icy crust on mud flows affects their rheology and their ability to spread across the surface. In addition, we do not know what the pressure gradient driving the flow of the mud up to the surface is, how a turbulent flow regime would affect the freezing rate of the mud, or when these mud-volcano-like features formed and what the conditions were like on the surface of Mars at the time. In summary, these gaps complicate the development of numerical models that would be capable of reconstructing the formation of martian sedimentary volcanoes and would enable us to study the role of individual parameters in sedimentary volcanic processes on Mars. In turn, these shortcomings limit our ability to predict the surface morphology of the resulting sedimentary edifices and hence what to search for.
From a general point of view, and in analogy with Earth, the initiation of sediment mobilisation on Mars requires several conditions to be met: (a) the relatively quick accumulation of sufficiently thick sedimentary deposits, (b) the presence of at least some strata of fine-grained sediments and liquid water within these deposits, and (c) overpressurisation of the sediments or gravitational instability as a trigger of fluid expulsion (and likely gas expulsion as well). In other words, sedimentary volcanism on Mars cannot occur anywhere, but should be restricted to specific locations where favourable conditions were present in the past.
As a first prerequisite for sediment mobilisation and MV, sizable depocentres must have been available that could be filled over time with sufficiently thick volatile-rich sedimentary strata. On Earth, such areas of sediment accumulation are often linked to plate boundaries (e.g. Dickinson, 1974; Miall, 1984), whereas Mars is a one-plate planet (Solomon, 1978) and does not show evidence of plate tectonics (Tosi and Padovan, 2021). Therefore, other suitable locations with sufficient sedimentary accumulation have to be selected. The ideal candidate locations displaying such characteristics were identified by many studies and include (a) large ancient impact basins that would have acted as sinks for water and sediments that were transported in giant flood events through outflow channels (e.g. McGill, 1989; Frey et al., 2002; Ivanov et al., 2014; Jones et al., 2016) together with their circumferential annular spaces (e.g. Skinner and Tanaka, 2007) and (b) large troughs created by extensional tectonics and/or collapse (notably Valles Marineris), some of which may have once hosted lakes (e.g. Harrison and Grimm, 2004; Harrison and Chapman, 2008; Warner et al., 2013; Okubo, 2016). For example, Tewelde and Zuber (2013) proposed that 2–4 km of sediment fill accumulated in Acidalia Planitia and up to 5 km of sediments were deposited in Utopia Planitia over time. However, at the moment, the exact sediment thicknesses and sedimentation/compaction rates in the basins remain unknown. For a rare study on sediment compaction on Mars, see Gabasova and Kite (2018); however, this study does not cover sedimentation in the large depocentres of the northern lowlands where most candidate mud volcanoes have been identified.
The second prerequisite is the infilling of such depocentres with fine-grained sediments that could be potentially mobilised. Several mechanisms have been proposed for the transport and deposition of such fine-grained sediments. For example, Okubo (2016) proposed that aeolian deposition within the Hesperian epoch might have partly infilled the Candor Chaos and Coprates Chasma regions and that these sediments might have been later buried by Middle Amazonian sediments and mobilised into large muddy laccoliths. Alternatively, Ivanov et al. (2014) proposed that fine-grained sediments might have originated from an ancient muddy ocean fed by outflow channels floods (see also Jöns, 1985). Similar outflow channel floods were also proposed as the source of fine-grained sediments within Chryse Planitia, as analogous to terrestrial outflow events (Rice and Edgett, 1997). An additional mechanism is the deposition of fine-grained volcanic ash originating from explosive volcanic eruptions (Ivanov et al., 2012), resulting in thick and extensive pyroclastic deposits deposited over volatile-rich units (see Brož et al., 2021, and references therein). These pyroclastic deposits could then be subsequently buried by younger material.
The third prerequisite is the generation of overpressure or gravitational instability required to mobilise sediment, water, and/or other volatiles. Hypotheses include (a) top-down freezing of water-bearing (i.e. muddy) sediment bodies, i.e. the gradual thickening of a cryosphere (Clifford et al., 2010; Ivanov et al., 2014; Ivanov and Hiesinger, 2020) and (b) rapid burial of sedimentary strata by mass-wasting processes like lahars, landslides, and impacts (e.g. Tanaka, 1999; Skinner and Tanaka, 2007; Skinner and Mazzini, 2009). Overpressure resulting from these types of processes may be (c) enhanced by gas released if clathrates are destabilised (as could occur by uplift, sublimation of a frozen ocean, temperature changes, etc.; e.g. Oehler and Allen, 2010; Oehler and Etiope, 2017), (d) seismic activity caused by internal martian processes or by impacts (e.g. Skinner and Tanaka, 2007), (e) rapid changes in the local tectonic regime (e.g. Hemmi and Miyamoto, 2017), or (f) combinations of these mechanisms. In addition, one must consider the possibility that a large quantity of clathrates may have been destabilised if, for example, a frozen ocean sublimated away. If that occurred, then clathrate destabilisation might be a major source of overpressure and MV in some areas. However, currently, there is neither ground truth nor numerical modelling available to assess such scenarios. In fact, is not even clear if gas is needed to drive MV on Mars.
Despite this uncertainty, it is reasonable to expect that one or more of these mechanisms were likely active in Mars' history. As individual martian sedimentary depocentres were subject to different geological settings and conditions and as the climate and aqueous activity on Mars has changed over time (see Sect. 3.4), possible sediment mobilisations did not necessarily happen during the same epochs. This could apply to both the time when the sediments were emplaced and when they were subsequently mobilised in the subsurface. It is most likely that water-rich sediments were emplaced when liquid water was more widespread on Mars than in the Middle to Late Amazonian. It seems reasonable to assume that the sediments therefore accumulated when the valley networks and, perhaps more importantly, the outflow channels were formed (Late Noachian–Early Hesperian and Late Hesperian–Early Amazonian respectively). As for the mobilisation, it is likely that most potential triggers for mobilisation would have been active throughout martian history (e.g. large impacts: Hartmann and Neukum, 2001; seismicity: Knapmeyer et al., 2006; note that the InSight seismometer has demonstrated present-day seismicity on Mars: for a recent review, see Lognonné et al., 2023). The thickening of the cryosphere (top-down freezing) is also probably an ongoing process, and may have been more important as a trigger of MV in the Hesperian and Amazonian than in the Noachian. As a result of such changes and the associated effects of the martian environment on mud behaviour (see Sect. 3.4), martian putative sedimentary volcanoes and mud flows might show large morphological and chronological variability. In fact, distinct depocentres would host differently sized mud reservoirs, with sediment of diverse lithologies and geochemical provenance, contain variable amounts of available water, and experience diverse conditions (e.g. overpressurisation, density stratification) that may have triggered sediment liquefaction and mobilisation. Variations within this parameter space could have controlled the effusion rates during mud expulsion as well as the water content within the mud, and in turn the viscosity of the ascending mixture might show a broad variability (e.g. Brož et al., 2019) resulting in a potentially large morphological diversity of sedimentary landforms (see previous section).
This review aims to outline the fundamental concepts and definitions of MV on Earth and to identify and describe martian examples of features that could be associated with a similar formation mechanism. The fundamental question is as follows: are these martian features analogous to terrestrial mud volcanoes? Or, conversely, is it impossible to provide a definitive answer based on our current knowledge? Throughout this review we have applied the term “mud-volcano-like” to structures that may have had a similar formation process to mud volcanoes on Earth. Considering the parameters that are commonly used to define MV-related phenomena on Earth, there are several aspects that need to be resolved and further investigated before we can apply Earth-based definitions to martian structures. Whereas the surface morphology of the investigated features is relatively well characterised at least at the metre to kilometre scale (Sect. 3), the subsurface mechanisms leading to sediment mobilisation and the link to tectonic settings remain unclear. We still cannot constrain the composition and origin of the mobilised sediments emplaced on the surface, the associated amount of water and gases, or the triggering mechanism that would initiate sedimentary volcanism on Mars. Section 2.4 underlined key questions that should be addressed to better assess the potential of MV on Mars.
Sedimentary deposits and structural discontinuities. Most of the largest terrestrial mud volcanoes are formed in areas of major compressional stress at plate boundaries, and the source areas are at kilometre-scale depths (Mazzini and Etiope, 2017). It is known that thick accumulations of layered sediments exist on Mars, and they can locally reach thicknesses of several kilometres (e.g. Malin and Edgett, 2000; Milliken et al., 2010). Although these deposits (e.g. in Valles Marineris or in Gale crater) are exposed at the surface and are most likely not a viable source of deep-seated MV, several ancient depocentres, especially in large ancient impact basins, are believed to host depositional units some kilometres thick at depth (e.g. Lucchitta et al., 1986; Goldspiel and Squyres, 1991) that could act as significant reservoirs of mobile and potentially buoyant sediments which ultimately could have erupted at the surface. At the termination of outflow channels, voluminous sediments must have been deposited by flooding events and reach minimum thicknesses of more than several hundred metres (e.g. Tanaka, 1997). Direct observational evidence of such deeply buried sediments is lacking, however, and multispectral analyses of materials excavated by impact crater formation in the northern lowlands do not strongly support thick deposits of sediments in the basins with the most numerous mud volcano candidates (Chryse, Acidalia, Utopia; Pan et al., 2017). Geophysical results are inconclusive, too. Although the average bulk density of the martian crust is lower than expected than for a mafic (basaltic) crust (Goossens et al., 2017) and lateral variations may exist, the average density seems to be lower in the southern highlands than in the northern lowlands (Wieczorek et al., 2022), which appears inconsistent with large volumes of low-density sediments in the lowlands (although it should be noted that the highest crustal densities in the lowlands correspond to volcanic provinces, not the large impact basins; Belleguic et al., 2005; Goossens et al., 2017). Nevertheless, recent seismic observations obtained through measurements by the InSight lander indicate lower shear-wave velocities in the lowlands as compared to the highlands, which could be due to thick accumulations of sediments (Li et al., 2022). New orbiters with powerful radar instruments capable of taking high-resolution gravity measurements (Genova, 2020; Oberst et al., 2022) would be essential to identify and characterise any voluminous wet sediments in the deep martian subsurface.
The importance of faults and other structural discontinuities in facilitating sediment/fluid flow on Earth was highlighted in Sect. 2. Mars has lacked plate tectonics for most (or possibly all) of its history (e.g. Grott et al., 2013, Smrekar et al., 2019, and references therein), and although poorly constrained, the martian subsurface is considered to be extensively faulted and fractured after a long history of impacts, the formation and evolution of the dichotomy, and the uplift of Tharsis (Golombek and Phillips, 2010). The northern lowland areas, where most of the candidate mud volcanoes are observed, are structurally dominated by wrinkle ridges, considered to be evidence of contractional deformation of a layered substrate (see review of Mueller and Golombek, 2004). Therefore, there are certainly tectonic discontinuities in the areas of possible sedimentary volcanism. However, they are mostly indicative of compressional stress, and the dip angle of the thrust faults associated with wrinkle ridges is relatively shallow (average ∼ 30∘, according to many models; e.g. Karagoz et al., 2022). Although there may be a large range of possible dips (see discussion by Andrews-Hanna, 2020), it is not clear whether these faults may have acted as preferential pathways for liquefied sediment.
Hydrocarbons. As explained in Sect. 2, MV on Earth is exclusively observed in hydrocarbon-bearing sedimentary basins and commonly associated with the release of significant volumes of methane (Mazzini and Etiope, 2017), a gas that significantly contributes to the greenhouse effect. At the moment, we do not know what type of gases (if any) may have been released if subsurface sediment mobilisation was ever active on Mars. A summary of current observations in that regard have been provided by Oehler and Etiope (2021). The authors discuss the potential of methane on Mars (with both abiotic and potential biotic sources assessed) as well as the discrepancies between the methane measurements by Curiosity (Webster et al., 2018) and the non-detection by the TGO (Korablev et al., 2019). Oehler and Etiope (2021) conclude that there is a strong case for the production of abiotic methane in the subsurface of Mars. Much of that could have been produced in the early history of the planet and may have already seeped to the surface. If so, remaining quantities are likely to be trapped by the planet-wide cryosphere, resulting in minor and episodic releases – potentially below the detection limits of the TGO. Nevertheless, methane may have been present in the martian subsurface in substantial amounts early in the planet's history. In addition, since the martian atmosphere is CO2 rich, methane and CO2 are potential gases that could have been released by sedimentary volcanism on Mars. If we assume that martian sedimentary volcanoes were indeed accompanied by large releases of methane (or other greenhouse gases), this would imply that those gases could have contributed to transitory climate warming in the past and supports the potential existence of methane reservoirs at depth (Oehler and Etiope, 2021). However, as no signs of active sedimentary volcanism on Mars have been discovered so far and as the ages of putative sedimentary edifices determined by crater counting suggest activity at least dozens or hundreds of millions of years ago (Brož and Hauber, 2013; Brož et al., 2017, 2019), it is therefore highly unlikely that ancient sedimentary volcanism is the source of martian atmospheric methane detected today (e.g. Giuranna et al., 2019; Oehler and Etiope, 2021, and references therein). Lefèvre and Forget (2009) showed that methane should have a relatively short lifetime in the current atmosphere of Mars – around 300 years in the upper atmosphere but only 200 d or less when close to the surface. Therefore, recent methane detections are likely to have been released by different mechanisms, e.g. by seepage from partly sealed subsurface reservoirs. As such, it now remains unclear if a genetic link between martian mud-volcano-like structures and methane releases exists at all.
Triggering mechanisms. On Earth, gravitational instability of shales and gas overpressure, as well as water present at buried deposits, are crucial factors promoting sediment mobilisation (Mazzini and Etiope, 2017). It is extremely unlikely that all of these mechanisms operated (or even operate) in the subsurface of Mars (e.g. Oehler and Allen, 2010) due to the likely absence of comparable quantities of hydrocarbons and plate tectonics. Other mechanisms (summarised in Sect. 4) have therefore been proposed. For example, it was proposed by Oehler and Etiope (2021) that clathrate destabilisation might account for the majority of the bright mounds in Acidalia where shallow-rooted conduits are suggested to follow a model similar to that recorded from Lake Baikal (Khlystov et al., 2019). If such a scenario is proven to be true, the vast number of these mounds would reveal the extent to which clathrates are buried across the martian northern lowlands.
However, as none of these scenarios are supported by ground truth evidence, physical models, or numerical modelling results, this limits our ability to understand the way sedimentary volcanism and the possible associated release of greenhouse gases would affect the evolution of Mars and hence to understand whether or not sedimentary volcanism could have helped to alter the atmosphere of early Mars (Wordsworth, 2016).
Currently available remote sensing data, despite their diversity and high spatial resolution, provide only limited insights into the nature of proposed candidate sedimentary volcanoes. The origin of a specific landform can be interpreted differently because of equifinality, a problem from which planetary geology suffers in the investigation of many surface features (e.g. Beven, 1996; Komatsu, 2007). Spectral data obtained from orbit are not conclusive either (e.g. Komatsu et al., 2016; Dapremont and Wray, 2021). A definitive proof of sedimentary volcanism on Mars is hampered by the lack of in situ data (ground truth) that could provide unambiguous evidence of their formation. Until now, in situ observations supporting the presence of subsurface sediment mobilisation are very limited. In this section, we describe the most promising examples that have been, or can be, visited on the martian surface and suggest tests of the sedimentary volcano hypothesis.
6.1 Sedimentary pipes in Gale crater
The Mars Science Laboratory mission with its rover, Curiosity, has traversed fluvio-lacustrine and aeolian sedimentary rocks that were deposited in the Gale crater ∼ 3.6 to 3.2 Ga (Rubin et al., 2016). Structures interpreted to be pipes formed by vertical movement of fluidised sediment were observed at several locations (Fig. 6a–d; Rubin et al., 2016). Circular rings of erosion-resistant material with diameters of 7 to 70 cm rise a few centimetres above their surroundings and display cementation and concentric internal layering. They are associated with other potential fluidised sediment features such as sedimentary dikes (Fig. 6e; Grotzinger et al., 2013) and deformational structures and may be analogues to clastic pipes on the Colorado Plateau (Ormö et al., 2004; Mahaney et al., 2004; Wheatley et al., 2016, 2019). Clastic pipes are injection features that vertically crosscut bedding with sharp contacts (Fig. 7). They display cylindrical morphologies, massive or radially graded interiors, and raised outer rims. Increased grain size and subsequent cementation along the more porous edges make the rims more resistant to weathering. Pipes have crosscutting relationships with other pipes due to multiple formation events or migrating eruption centres, and they are associated with other soft-sediment deformation features. Terrestrial clastic pipes form via liquefaction and fluidisation, which require a near-surface groundwater system to initiate (e.g. Jamtveit et al., 2004; Svensen et al., 2006).
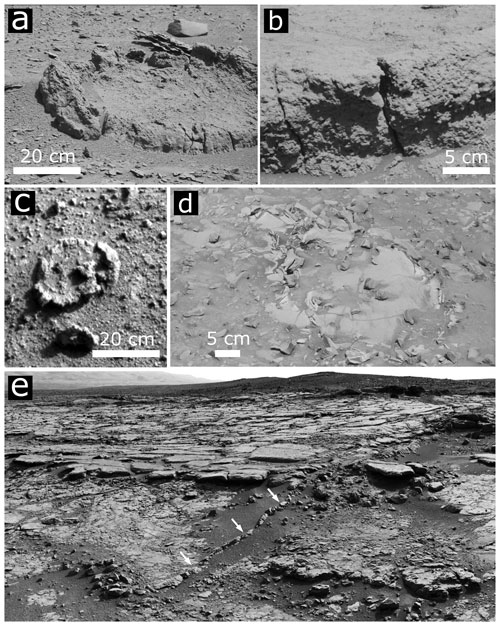
Figure 6Examples of outcrop features observed by the Curiosity rover inside Gale crater on Mars hypothesised to have formed by the upward injection of sediment through the crust. Panel (a–d): various pipes observed at the Dingo Gap and Marias Pass. Figures are adapted from Rubin et al. (2016). Panel (e): dike observed at Yellowknife Bay (arrows). Image numbers: (a) 0528MR0020830010303294E01_DXXX, (b) MR002083, (c) NLB_445620806EDR_F0261274NCAM00354M, (d) 1051ML
0046250040306086E01_DXXX, and (e) 20170206PIA17595-16. NASA/JPL-Caltech/MSSS.
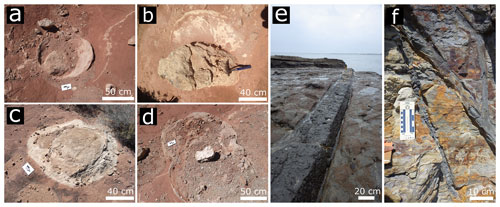
Figure 7Terrestrial analogues for the martian outcrop features hypothesised to have formed by upward injection of sediment. (a–d) Clastic pipes widely observed within the Colorado Plateau. (e, f) Sedimentary dikes exposed horizontally (e; the dike width is about 30 cm) and vertically (f) along the western coast of the Kii Peninsula. These dikes resulted from an ancient (as old as the Miocene) process of subsurface sediment mobilisation. Photos in (a–d) by © David Wheatley (all rights reserved) and (e, f) by Goro Komatsu.
Another potentially interesting and relevant analogue is identified in western Japan along the Kii Peninsula coast, where ancient MV is preserved and exposed in sedimentary sequences (Komatsu et al., 2019). Coarse-grained shallow marine sediment sequences of the Miocene are intruded upon by the underlying fine-grained sediment. The intruding mudstone deposits exhibit diverse types of stratigraphic features, including mud dikes intruding into overlying layers or diapirs in contact with the surrounding strata (Fig. 7). Like many pipes on Earth, the structures in Gale crater are more resistant to erosion than the host rock; they form near other pipes, dikes, or deformed sediment; some contain internal concentric or eccentric layering. These structures provide new evidence of the importance of subsurface aqueous processes in shaping the near-surface geology of Mars (Rubin et al., 2016).
6.2 Zhurong rover study of one putative martian sedimentary volcano
The Chinese Zhurong rover onboard Tianwen-1 landed on southern Utopia Planitia (25.066∘ N, 109.925∘ E) on 15 May 2021. In the vicinity of the landing site, orbital imagery revealed the presence of a field of cone-shaped edifices (Liu et al., 2021; Ye et al., 2021; Zhao et al., 2021). In the field, there is one pitted cone with a height of 80 m and a basal diameter of 800 m located about 16 km south-east of the landing site, and it has attracted the attention of the mission science team (Liu et al., 2021). Alternative interpretations, including cinder cones, sedimentary volcanoes, or pingos, have been proposed to explain the origin of this structure. Sedimentary volcanism appears to be the preferred origin as reported in recent studies (Ye et al., 2021; Huang et al., 2022), although other small mounds in the region have been interpreted as lava domes (Lin et al., 2023). In situ study of the closest cone to the landing site would provide a great opportunity for ground-truthing one example of putative sedimentary volcanoes on Mars. The identification of clay minerals, like smectite or illite (Mazzini and Etiope, 2017, and references therein), as a main bulk component of the pitted cones would be strong evidence of a mud volcano origin.
6.3 Future in situ investigation of hypothesised sedimentary volcanism
Some proposals have been made regarding the importance of in situ investigation of purported sedimentary volcanism (Komatsu et al., 2014), and some candidate landing sites can be listed at some areas of hypothesised sedimentary volcanism such as those in Chryse Planitia (Rodriguez et al., 2007; Komatsu et al., 2011, 2016; Brož et al., 2019; Komatsu and Brož, 2021).
Conceivable in situ investigations at a future landing site may include those for (a) geology, (b) geo-biochemistry, and (c) biology. Firstly, the origin of the edifices must be investigated. In situ lithological and mineralogical examination of edifices' surface or subsurface would be essential to distinguish the formation processes. A confirmed sedimentary volcanism scenario would bring insights about the water occurrence both on the surface and in the subsurface of Mars. Further, an analysis of the erupted mud breccia clasts would provide unprecedented information regarding the subsurface geology at these sites.
On Earth, mud volcanoes host a large variety of microbial communities that thrive particularly at seepage sites (e.g. Wrede et al., 2012; Kokoscha et al., 2015; Tu et al., 2017; Lee et al., 2021; Miyake et al., 2023). It has even been suggested that fluids ascending from deeply subducted slabs may have led to low-temperature alteration environments in the conduits of serpentinite mud volcanoes that provided suitable niches for early life (e.g. Pons et al., 2011; Fryer, 2012; Fryer et al., 2020). The study of putative mud-volcano-like structures has therefore great potential for astrobiology investigation and for the collection of fossilised microorganisms (e.g. Komatsu and Ori, 2000). Such investigation of martian sedimentary volcanoes should focus on localities where mud eruptions occurred, including summit craters and small mud mounds (called gryphons) where emissions of mud and gas might continue for a prolonged period of time even after major eruptions. However, young, fresh-looking mudflows emanating away from the summit craters are also promising candidate targets. It is recommended to conduct drilling ≥ 2 m into the mud in order to sample materials less exposed to the harsh surface environment and protected from surface radiation (Pavlov et al., 2022). The only currently existing rover equipped to drill to that depth is the ExoMars rover (Vago et al., 2017), which is planned to land in the Oxia Planum region in 2031. While the main targets are phyllosilicate-bearing layered deposits, there are some kilometre-sized mounds in the area that form part of a regional population of mounds in the southern and eastern marginal regions of the Chryse impact basin (McNeil et al., 2021). Some of these mounds have been interpreted to be products of sedimentary volcanism (Adler et al., 2022), and if the ExoMars rover lands near one of these mounds, it is recommended that it investigates it in situ.
After several decades of Mars exploration, there is a growing consensus that a phenomenon similar to sedimentary volcanism on Earth may have been active on the Red Planet. An improved understanding of martian geological settings and higher-quality remote sensing images have helped in assessing the origin (sedimentary versus igneous) of numerous enigmatic features observed on the surface. It remains so far unresolved if many of the observed martian features are the result of mechanisms similar in some aspects to those triggering mud volcano activity on Earth. As described herein, MV on Earth has very particular constraints. However, we do not know whether those constraints exist on Mars. For these reasons we suggest the use of the “mud-volcano-like” terminology. This paper reviews the martian regions where mud-volcano-like features occur and provides detailed descriptions of the observed morphologies and the proposed formation mechanisms.
The greatest abundance of mud-volcano-like features occurs in the northern lowlands of Mars, commonly north of the latitudinal maximum limit that has governed the landing-site selection for previous landed missions. The largest abundances are in Acidalia and Utopia planitiae, where a hundred thousand of such structures occur, but examples also occur in Chryse, Arcadia, and Isidis planitiae. Outside the northern lowlands, only a few fields of such mounds have been identified, for example in Arabia Terra, Valles Marineris, and Terra Sirenum. These numerous mounds clearly reflect major events in the history of the martian lowlands – events that could have initiated warming episodes and may even have global climatic relevance. In addition to these numerous, near-circular edifices, larger and morphologically/morphometrically more diverse features of similar origin have been also discovered. They are represented by kilometre-scale edifices with cross-sectional shapes of domes, shields, and flow-like features with lengths of up to dozens of kilometres.
As outlined in this overview, there should be favourable conditions in the subsurface of Mars enabling the process of sediment mobilisation and hence sedimentary volcanism. This is because thick accumulations of layered sediments exist on this planet that act as significant reservoirs of potentially mobile and buoyant sediments; at the same time, these sediments might be extensively faulted and fractured, enabling mobilised sediment to propagate to the surface. However, it is currently unknown what the exact mechanism responsible for such mobilisation would be, because we do not know what type of gases (if any) may have been released. Therefore, whether the process of subsurface sediment mobilisation on Mars is an exact analogue of the process of terrestrial MV, which is governed by gas emissions, remains unsolved.
If the described mud-volcano-like structures on Mars share a formation mechanism similar to that observed on Earth, they would represent excellent targets for future landed missions, as they could archive unaltered biosignatures if life ever developed on Mars (Oehler and Etiope, 2021). On Earth, mud volcanoes are localities where methane is continuously released and represent ideal niches for habitability (e.g. Knittel and Boetius, 2009). They also provide a window into deep biosphere potential sedimentary strata (e.g. Plümper et al., 2017), as mud volcanoes bring sediments from metres to kilometres of depth to the surface via a relatively low temperature and pressure processes (i.e. unlike impact ejecta that are associated with high pressure and temperature). The study of similar features on Mars could, thus, provide insightful information about currently inaccessible buried stratigraphy.
The Chinese rover Zhurong landed near a potential mud-volcano-like mound in Utopia Planitia, which may have provided an unprecedented opportunity to collect important observations about the origin of the material(s) forming the edifice. Dedicated future missions (e.g. using drones or helicopters) should focus on sample analysis or even sample collection from some of the potential sedimentary volcanoes in the northern latitudes. In parallel, work on analogue experiments through laboratory simulations of mud flows under Mars-like conditions and the associated numerical models will help to assess the origin and significance of some of the various edifices identified on Mars. Future Mars exploration should therefore plan efforts to investigate these enigmatic features, which could be excellent candidates for missions aimed at biosignature detection.
All data used in this study can be found in the Supplement.
The supplement related to this article is available online at: https://doi.org/10.5194/esurf-11-633-2023-supplement.
All co-authors contributed to the preparation of the article and the individual sections were greatly enhanced by interactions and discussions between the authors. PB and EH were the main authors of Sects. 1 and 4; AM and GE of Sect. 2; DO of Sects. 3.1.1 and 3.2; PB of Sects. 3.1.2, 3.3, and 3.4; VC of Sect. 3.1.3; PB, EH, and AM of Sect. 5; and GK of Sect. 6. Section 7 is then the result of the joint efforts of all co-authors.
The contact author has declared that none of the authors has any competing interests.
Publisher's note: Copernicus Publications remains neutral with regard to jurisdictional claims in published maps and institutional affiliations.
This article is part of the special issue “Planetary landscapes, landforms, and their analogues”. It is not associated with a conference.
We are thankful to David Wheatley for providing us with images of the clastic pipes found in Fig. 7 and to Dave Rubin for helping us locate the martian images used in Fig. 6. We would also like to thank Jim Skinner and an anonymous referee for their constructive comments and Susan J. Conway for handling the editorial process. Adriano Mazzini acknowledges the support of the Research Council of Norway (NFR) through HOTMUD project no. 288299. Vojtěch Cuřín acknowledge the support of the IGA Faculty of Environmental Sciences CZU Prague through the grant “Kilometre-Sized Flows on Mars: Controlling Factors and Geomorphology – no. 2022B0038”.
This research has been supported by the Norges Forskningsråd (grant no. 288299).
This paper was edited by Susan Conway and reviewed by James Skinner Jr. and one anonymous referee.
Adler, J. B., Bell, J. F., Warner, N. H., Dobrea, E. N., and Harrison, T. N.: Regional geology of the Hypanis Valles system, Mars, J. Geophys. Res.-Planet., 127, e2021JE006994, https://doi.org/10.1029/2021JE006994, 2022.
Akhmanov, G. G., Premoli Silva, I., Erba, E., and Cita, M. B.: Sedimentary succession and evolution of the Mediterranean Ridge western sector as derived from lithology of mud breccia clasts, Mar. Geol., 195, 277–299, https://doi.org/10.1016/S0025-3227(02)00693-X, 2003.
Alemanno, G., Orofino, V., and Mancarella, F.: Global map of Martian fluial systems: Age and total eroded volume estimations, Earth Space Sci., 5, 560–577, https://doi.org/10.1029/2018EA000362, 2018.
Allen, C. C.: Volcano/ice interactions on Mars, J. Geophys. Res., 84, 8048–8059, https://doi.org/10.1029/JB084iB14p08048, 1979.
Aliyev, A., Guliyev, F., Dadashev, F. G., and Rahmannov, R. R.: Atlas of the world mud volcanoes, Nafta-Press, ISBN 978-9952-437-60-7, 2015.
Allen, C. C., Oehler, D. Z., and Baker, D. M.: Mud volcanoes – A new class of sites for geological and astrobiological exploration of Mars, in: 40th Lunar and Planetary Science Conference, 23–27 March 2009, The Woodlands, TX, Abs. #1749, 2009.
Allen, C. C., Oehler, D., Etiope, G., Van Rensbergen, P., Baciu, C., Feyzullayev, A., Martinelli, G., Tanaka, K., and Van Rooij, D.: Fluid expulsion in terrestrial sedimentary basins: a process providing potential analogs for giant polygons and mounds in the martian lowlands, Icarus, 224, 424–432, https://doi.org/10.1016/j.icarus.2012.09.018, 2013.
Andreassen, K., Hubbard, A., Winsborrow, M., Patton, H., Vadakkepuliyambatta, S., Plaza-Faverola, A., Gudlaugsson, E., Serov, P., Deryabin, A., Mattingsdal, R., Mienert, J., and Bünz, S.: Massive blow-out craters formed by hydrate-controlled methane expulsion from the Arctic seafloor, Science, 356, 6341, 948–953, https://doi.org/10.1126/science.aal4500, 2017.
Andrews-Hanna, J. C.: The tectonic architecture of wrinkle ridges on Mars, Icarus, 351, 113937, https://doi.org/10.1016/j.icarus.2020.113937, 2020.
Baker, V. R. and Milton, D. J.: Erosion by catastrophic floods on Mars and Earth, Icarus, 23, 27–41, https://doi.org/10.1016/0019-1035(74)90101-8, 1974.
Baker, V. R., Hamilton, C. W., Burr, D. M., Gulick, V., Komatsu, G., Luo, W., Rice Jr., J. W., and Rodríguez, J. A. P.: Fluvial geomorphology on Earth-like planetary surfaces: a review, Geomorphology 245, 149–182, https://doi.org/10.1016/j.geomorph.2015.05.002, 2015.
Bargery, A. S., Lane, S. J., Barrett, A., Wilson, L., and Gilbert, J. S.: The initial responses of hot liquid water released under low atmospheric pressures: experimental in-sights, Icarus, 210, 488–506, https://doi.org/10.1016/j.icarus.2010.06.019, 2010.
Belleguic, V., Lognonné, P., and Wieczorek, M.: Constraints on the Martian lithosphere from gravity and topography data, J. Geophys. Res., 110, E11005, https://doi.org/10.1029/2005JE002437, 2005.
Beven, K.: Equifinality and uncertainty in geomorphological modelling, in: The Scientific Nature of Geomorphology, in: Proc. 27th Binghampton Symp. Geomorphol., 27–29 September 1996, Binghamton, edited by: Rhoads, B. L. and Thorn, C. E., Wiley, Chichester, 289–313, 1996.
Bleacher, J. E., Greeley, R., Williams, D. A., Cave, S. R., and Neukum, G.: Trends in effusive style at the Tharsis Montes, Mars, and implications for the development of the Tharsis province, J. Geophys. Res., 112, E09005, https://doi.org/10.1029/2006JE002873, 2007.
Bouriak, S., Vanneste, M., and Saoutkine, A.: Inferred gas hydrates and clay diapirs near the Storegga Slide on the southern edge of the Vøring Plateau, offshore Norway, Mar. Geol., 163, 1–4, 125–148, https://doi.org/10.1016/S0025-3227(99)00115-2, 2000.
Brass, G. W.: Stability of brines on Mars, Icarus, 42, 20–28, https://doi.org/10.1016/0019-1035(80)90237-7, 1980.
Bristow, C. R., Gale, I. N., Fellman, E., Cox, B. M., Wilkinson, I. P., and Riding, J. B.: The lithostratigraphy, biostratigraphy and hydrogeological significance of the mud springs at Templars Firs, Wootton Bassett, Wiltshire, Proceedings of the Geologists' Association, 111, 231–245, https://doi.org/10.1016/S0016-7878(00)80016-4, 2000.
Brož, P. and Hauber, E.: Hydrovolcanic tuff rings and cones as indicators for phreatomagmatic explosive eruptions on Mars, J. Geophys. Res., 118, 1656–1675, https://doi.org/10.1002/jgre.20120, 2013.
Brož, P., Čadek, O., Hauber, E., and Rossi, A. P.: Scoria cones on Mars: detailed investigation of morphometry based on high-resolution digital elevation models, J. Geophys. Res., 120, 1512–1527, https://doi.org/10.1002/2015JE004873, 2015a.
Brož, P., Hauber, E., Platz, T., and Balme, M. R.: Evidence for Amazonian highly viscous lavas in the southern highlands on Mars, Earth Planet. Sc. Lett., 415, 200–212, https://doi.org/10.1016/j.epsl.2015.01.033, 2015b.
Brož, P., Hauber, E., Wray, J. J., and Michael, G.: Amazonian volcanism inside Valles Marineris on Mars, Earth Planet. Sc. Lett., 473, 122–130, https://doi.org/10.1016/j.epsl.2017.06.003, 2017.
Brož, P., Hauber, E., van de Burgt, I., Špillar, V., and Michael, G.: Subsurface sediment mobilization in the southern Chryse Planitia on Mars, J. Geophys. Res., 124, 703–720, https://doi.org/10.1029/2018JE005868, 2019.
Brož, P., Krýza, O., Wilson, L., Conway, S. J., Hauber, E., Mazzini, A., Raack, J., Patel, M. R., Balme, M. R., and Sylvest, M. E.: Experimental evidence for lava-like mud flows under Martian surface conditions, Nat. Geosci., 13, 403–407, https://doi.org/10.1038/s41561-020-0577-2, 2020a.
Brož, P., Krýza, O., Conway, S. J., Mueller, N. T., Hauber, E., Mazzini, A., Raack, J., Patel, M. R., Balme, M. R., and Sylvest, M. E.: Mud flow levitation on Mars: insights from laboratory simulations, Earth Planet. Sc. Lett., 545, 116406, https://doi.org/10.1016/j.epsl.2020.116406, 2020b.
Brož, P., Bernhardt, H., Conway, S. J., and Parekh, R.: An overview of explosive volcanism on Mars, J. Volcanol. Geoth. Res., 409, https://doi.org/10.1016/j.jvolgeores.2020.107125, 2021.
Brož, P., Hauber, E., Conway, S. J., Luzzi, E., Mazzini, A., Noblet, A., Jaroš, J., Fawdon, P., and Markonis, Y.: New Evidence for Sedimentary Volcanism on Chryse Planitia, Mars, Icarus, 382, 115038, https://doi.org/10.1016/j.icarus.2022.115038, 2022a.
Brož, P., Krýza, O., Conway, S. J., Mazzini, A., Hauber, E., Sylvest, M. E., and Patel, M. R.: Volumetric changes of mud on Mars: evidence from laboratory simulations, in: 53rd lunar and Planetary science conference, 7–11 March 2022, The Woodlands, TX, #1337, 2022b.
Bulanova, I. A., Solovyeva, M. A., Akhmanov, G. G., Khlystov, O. M., and Starovoytov, A. V.: Results of geological and geophysical studies of the Elovsky (Lake Baikal), Proceedings of VII International conference “Marine Research and Education (MARESEDU-2018)” Moscow, 19–22 November 2018, v. 2, no. LLC “PolyPRESS”, Tver', 2019, p. 153–154, 2018.
Calvari, S. and Pinkerton, H.: Lava tube morphology on Etna and evidence for lava flow emplacement mechanisms, J. Volcanol. Geoth. Res., 90, 263–280, https://doi.org/10.1016/S0377-0273(99)00024-4, 1999.
Carr, M. H.: Stability of streams and lakes on Mars, Icarus, 56, 476–495, https://doi.org/10.1016/0019-1035(83)90168-9, 1983.
Chan, M. A., Ormo, J., Murchie, S., Okubo, C. H., Komatsu, G., Wray, J. J., McGuire, P., and McGovern, J. A.: Geomorphic knobs of Candor Chasms, Mars: new Mars Reconnaissance Orbiter data and comparisons to terrestrial analogs, Icarus, 205, 138–153, https://doi.org/10.1016/j.icarus.2009.04.006, 2010.
Ciotoli, G., Procesi, M., Etiope, G., Fracassi, U., and Ventura, G.: Influence of tectonics on global scale distribution of geological methane emissions, Nat. Commun., 11, 2305, https://doi.org/10.1038/s41467-020-16229-1, 2020.
Cita, M. B., Ryan, W. B. F., and Paggi, L.: Prometheus mudbreccia: An example of shale diapirism in the Western Mediterranean Ridge, Ann. Geol. Pays Hellen., 30, 543–570, 1981.
Clifford, S. M., Lasue, J., Heggy, E., Boisson, J., McGovern, P., and Max, M. D.: Depth of the Martian cryosphere: Revised estimates and implications for the existence and detection of subpermafrost groundwater, J. Geophys. Res., 115, E07001, https://doi.org/10.1029/2009JE003462, 2010.
Cockell, C. S.: Trajectories of Martian Habitability, Astrobiology, 14, 182–203, https://doi.org/10.1089/ast.2013.1106, 2014.
Cockell, C. S. and Barlow, N.: Impact excavation and the search for subsurface life on Mars, Icarus, 155, 340–349, https://doi.org/10.1006/icar.2001.6725, 2002.
Conway, S. J., Lamb, M. P., Balme, M. R., Towner, M. C., and Murray, J. B.: Enhanced runout and erosion by overland flow at low pressure and subfreezing conditions: Experiments and application to Mars, Icarus, 211, 443–457. https://doi.org/10.1016/j.icarus.2010.08.026, 2011.
Cooper, C.: Mud volcanoes of Azerbaijan visualized using 3D seismic depth cubes: the importance of overpressured fluid and gas instead of non extant diapirs, Abstract Vol. Subsurface Sediment Mobilization Conf, 10–13 September, Ghent, Belgium, p. 71, 2001.
Costard, F., Séjourné, A., Jelloun, K., Clifford, S., Lavigne, F., Di Pietro, I., and Souley, S.: Modeling tsunami propagation and the emplacement of thumbprint terrain in an early Mars ocean, J. Geophys. Res.-Planet., 122, 633–649, https://doi.org/10.1002/2016JE005230, 2017.
Cuřín, V., Brož, P., Hauber, E., and Markonis, Y.: Mud flows in Southwestern Utopia Planitia, Mars, Icarus, 389, 115266, https://doi.org/10.1016/j.icarus.2022.115266, 2023.
Dapremont, A. M. and Wray, J. J.: Insights into Mars mud volcanism using visible and near-infrared spectroscopy, Icarus, 359, 114299, https://doi.org/10.1016/j.icarus.2020.114299, 2021.
Davis, P. A. and Tanaka, K. L.: Curvilinear ridges in Isidis Planitia, Mars – The result of mud volcanism?, in: 24th Lunar and Planetary Science, 15–19 March 1993, The Woodlands, TX, 321–322, 1995.
Dehn, J. and Sheridan, M. F.: Cinder cones on the Earth, Moon, Mars, and Venus: A computer model, in: 21st Lunar and Planetary Science Conference, 12–16 March 1990, The Woodlands, TX, #270, 1990.
De Toffoli, B., Pozzobon, R., Massironi, M., Mazzarini, F., Conway, S., and Cremonese, G.: Surface expressions of subsurface sediment mobilization rooted into a gas hydrate-rich cryosphere on Mars, Sci. Rep.-UK, 9, 8603, https://doi.org/10.1038/s41598-019-45057-7, 2019.
De Toffoli, B., Massironi, M., Mazzarini, F., and Bistacchi, A.: Rheological and mechanical layering of the crust underneath thumbprint terrains in Arcadia Planitia, Mars, J. Geophys. Res.-Planet., 126 (11), https://doi.org/10.1029/2021JE007007, 2021.
Dickinson, W. R.: Tectonics and Sedimentation, SEPM Special Publication, Vol. 22, SEPM Society for Sedimentary Geology, https://doi.org/10.2110/pec.74.22, 1974.
Dimitrov, L. I.: Mud volcanoes–the most important pathway for degassing deeply buried sediments, Earth Sci. Rev., 59, 49–76, https://doi.org/10.1016/S0012-8252(02)00069-7, 2002.
Dimitrov, L. I.: Mud volcanoes—a significant source of atmospheric methane, Geo-Mar. Lett., 23, 155–161, https://doi.org/10.1007/s00367-003-0140-3, 2003.
Di Pietro, I., Séjourné, A., Costard, F., Ciazela, J., and Rodríguez, A. P.: Evidence of mud volcanism due to the rapid compaction of martian tsunami deposits in southeastern Acidalia Planitia, Mars, Icarus, 354, 114096, https://doi.org/10.1016/j.icarus.2020.114096, 2021.
Etiope, G. and Milkov, A. V. : A new estimate of global methane flux from onshore and shallow submarine mud volcanoes to the atmosphere, Environ. Geol., 46, 997–1002, https://doi.org/10.1007/s00254-004-1085-1, 2004.
Etiope, G., Feyzullayev, A., and Baciu, C. L.: Terrestrial methane seeps and mud volcanoes: a global perspective of gas origin, Mar. Petrol. Geol., 26, 333–344, https://doi.org/10.1016/j.marpetgeo.2008.03.001, 2009.
Etiope, G., Baciu, C., and Schoell, M.: Extreme methane deuterium, nitrogen and helium enrichment in natural gas from the Homorod seep (Romania), Chem. Geol., 280, 89–96, https://doi.org/10.1016/j.chemgeo.2010.10.019, 2011.
Farrand, W. H., Gaddis, L. R., and Keszthlyi, L.: Pitted cones and domes on Mars: Observations in Acidalia Planitia and Cydonia Mensae using MOC, THEMIS, and TES data, J. Geophys. Res., 110, E05005, https://doi.org/10.1029/2004JE002297, 2005.
Formisano, V., Atreya, S., Encrenaz, T., Ignatiev, N., and Giuranna, M.: Detection of Methane in the Atmosphere of Mars, Science, 306, 1758–1761, https://doi.org/10.1126/science.1101732, 2004.
Franchi, F., Rossi, A. P., Pondrelli, M., and Cavalazzi, B.: Geometry, stratigraphy and evidences for fluid expulsion within Crommelin crater deposits, Arabia Terra, Mars, Planet. Space Sci., 92, 34–48, https://doi.org/10.1016/j.pss.2013.12.013, 2014.
Frey, H. M., Lowry, B. L., and Chase, S. A.: Pseudocraters on Mars, J. Geophys. Res., 84, 8075–8086, https://doi.org/10.1029/JB084iB14p08075, 1979.
Frey, H. V., Roark, J. H., Shockey, K. M., Frey, E. L., and Sakimoto, S. E. H.: Ancient lowlands on Mars, Geophys. Res. Lett., 29, 1384. https://doi.org/10.1029/2001GL013832, 2002.
Fryer, P.: Serpentinite Mud Volcanism: Observations, Processes, and Implications, Annu. Rev. Mar. Sci., 4, 345–373, https://doi.org/10.1146/annurev-marine-120710-100922, 2012.
Fryer, P., Wheat, C. G., Williams, T., Kelley, C., Johnson, K., Ryan, J., Kurz, W., Shervais, J., Albers, E., Bekins, B., Debret, B., Deng, J., Dong, Y., Eickenbusch, P., Frery, E., Ichiyama, Y., Johnston, R., Kevorkian, R., Magalhaes, V., Mantovanelli, S., Menapace, W., Menzies, C., Michibayashi, K., Moyer, C., Mullane, K., Park, J.-W., Price, R., Sissmann, O., Suzuki, S., Takai, K., Walter, B., Zhang, R., Amon, D., Glickson, D., and Pomponi. S.: Mariana serpentinite mud volcanism exhumes subducted seamount materials: implications for the origin of life, Philos. T. R. Soc. A, 378, 20180425, https://doi.org/10.1098/rsta.2018.0425, 2020.
Gabasova, L. R. and Kite, E. S.: Compaction and sedimentary basin analysis on Mars, Planet. Space Sci., 152, 86–106, https://doi.org/10.1016/j.pss.2017.12.021, 2018.
Gallagher, C., Balme, M., Soare, R., and Conway, S. J.: Formation and degradation of chaotic terrain in the Galaxias regions of Mars: implications for near-surface storage of ice, Icarus, 309, 69–83, https://doi.org/10.1016/j.icarus.2018.03.002, 2018.
Genova, A.: ORACLE: A mission concept to study Mars' climate, surface and interior, Acta Astronaut., 166, 317–329, https://doi.org/10.1016/j.actaastro.2019.10.006, 2020.
Gill, W. D. and Kuenen, P. H.: Sand volcanoes on slumps in the Carboniferous of County Clare, Ireland, Quarterly Journal of the Geological Society, 113, 441–460, https://doi.org/10.1144/GSL.JGS.1957.113.01-04.19, 1957.
Giuranna, M., Viscardy, S., Daerden, F., Neary, L., Etiope, G., Oehler, D., Formisano, V., Aronica, A., Wolkenberg, P., Aoki, S., Cardesin-Moinelo, A., Marin-Yaseli de la Parra, J., Merritt, D., and Amoroso, M.: Independent confirmation of a methane spike on Mars and a source region east of Gale Crater, Nat. Geosci., 12, 326–332, https://doi.org/10.1038/s41561-019-0331-9, 2019.
Goldspiel, J. M. and Squyres, S. W.: Ancient aqueous sedimentation on Mars, Icarus, 89, 392–410, https://doi.org/10.1016/0019-1035(91)90186-W, 1991.
Golombek, M. P. and Phillips, R. J.: Mars Tectonics, Chapter 5 in: Planetary Tectonics, edited by: Watters, T. R. and Schultz, R. A., Cambridge University Press, Cambridge, UK, 183–232, https://doi.org/10.1017/CBO9780511691645.006, 2010.
Goossens, S., Sabaka, T. J., Genova, A., Mazarico, E., Nicholas, J. B., and Neumann, G. A.: Evidence for a low bulk crustal density for Mars from gravity and topography, Geophys. Res. Lett., 44, 7686–7694, https://doi.org/10.1002/2017GL074172, 2017.
Grenfell, J. L., Wunderlich, F., Sinnhuber, M., Herbst, K., Lehmann, R., Scheucher, M., Gebauer, S., Arnold, G., and Rauer H.: Atmospheric processes affecting methane on Mars, Icarus, 382, 114940, https://doi.org/10.1016/j.icarus.2022.114940, 2022.
Grott, M., Baratoux, D., Hauber, E., Sautter, V., Mustard, J., Gasnault, O., Ruff, S. W., Karato, S.-I., Debaille, V., Knapmeyer, M., Sohl, F., Van Hoolst, T., Breuer, D., Morschhauser, A., and Toplis, M. J.: Long-term evolution of the Martian crust-mantle system, Space Sci. Rev., 174, 49–111, https://doi.org/10.1007/s11214-012-9948-3, 2013.
Grotzinger, J. P., Sumner, D. Y., Kah, L. C., Stack, K., Gupta, S., Edgar, L., Rubin, D., Lewis, K., Schieber, J., Mangold, N., Milliken, R., Conrad, P. G., DesMarais, D., Farmer, J., Siebach, K., Calef, F., Hurowitz, J., McLennan, S. M., Ming, D., Vaniman, D., Crisp, J., Vasavada, A., Edgett, K. S., Malin, M., Blake, D., Gellert, R., Mahaffy, P., Wiens, R. C., Maurice, S., Grant, J. A., Wilson, S., Anderson, R. C., Beegle, L., Arvidson, R., Hallet, B., Sletten, R. S., Rice, M., Bell, J., Griffes, J., Ehlmann, B., Anderson, R. B., Bristow, T. F., Dietrich, W. E., Dromart, G., Eigenbrode, J., Fraeman, A., Hardgrove, C., Herkenhoff, K., Jandura, L., Kocurek, G., Lee, S., Leshin, L. A., Leveille, R., Limonadi, D., Maki, J. , McCloskey, S., Meyer, M., Minitti, M., Newsom, H., Oehler, D., Okon, A., Palucis, M., Parker, T., Rowland, S., Schmidt, M., Squyres, S., Steele, A., Stolper, E., Summons, R., Treiman, A., Williams, R., Yingst, A., and MSL Science Team: A habitable fluvio-lacustrine environment at Yellowknife Bay, Gale Crater, Mars, Science, 343, 1242777, https://doi.org/10.1126/science.1242777, 2013.
Guest, J. E., Butterworth, P. S., and Greeley, R.: Geological observations in the Cydonia Region of Mars from Viking, J. Geophys. Res., 82, 4111–4120, https://doi.org/10.1029/JS082i028p04111, 1977.
Guliyev, I. S. and Feizullayev, A. A.: All about Mud volcanoes, Nafta Press, Baku, p. 52, ISBN 1801039000, 1997.
Haberle, R. M., McKay, C. P., Schaeffer, J., Cabrol, N. A., Grin, E. A., Zent, A. P., and Quinn, R.: On the possibility of liquid water on present-day Mars, J. Geophys. Res., 106, 23317–23326, https://doi.org/10.1029/2000JE001360, 2001.
Haberle, R. M., Catling, D. C., Carr, M. H., and Zahnle, K. J.: The Early Mars Climate System, in: The Atmosphere and Climate of Mars, edited by: Haberle, R. M., Clancy, R. T., Forget, F., Smith, M. D., and Zurek, R. W., Cambridge University Press, 526–568, https://doi.org/10.1017/9781139060172.017, 2017.
Harrison, K. P. and Chapman, M. G.: Evidence for ponding and catastrophic floods in central Valles Marineris, Mars, Icarus, 198, 351–364, https://doi.org/10.1016/j.icarus.2008.08.003, 2008.
Harrison, K. P. and Grimm, R. E.: Tharsis recharge: a source of groundwater for Martian outflow channels, Geophys. Res. Lett., 31, L14703, https://doi.org/10.1029/2004GL020502, 2004.
Hartmann, W. K. and Berman, D. C.: Elysium Planitia lava flows: Crater count chronology and geological implications, J. Geophys. Res., 105, 15011–15025, https://doi.org/10.1029/1999JE001189, 2000.
Hartmann, W. K. and Neukum, G.: Cratering chronology and the evolution of Mars, Space Sci. Rev., 96, 165–194, https://doi.org/10.1023/A:1011945222010, 2001.
Hauber, E., Bleacher, J., Gwinner, K., Williams, D., and Greeley, R.: The topography and morphology of low shields and associated landforms of plains volcanism in the Tharsis region of Mars, J. Volcanol. Geoth. Res., 185, 69–95, https://doi.org/10.1016/j.jvolgeores.2009.04.015, 2009.
Hauber, E., Brož, P., Jagert, F., Jodłowski, P., and Platz, T.: Very recent and widespread basaltic volcanism on Mars, Geophys. Res. Lett., 28, L10201, https://doi.org/10.1029/2011GL047310, 2011.
Hecht, M. H.: Metastability of liquid water on Mars, Icarus, 156, 373–386, https://doi.org/10.1006/icar.2001.6794, 2002.
Hemmi, R. and Miyamoto, H.: Distribution, morphology, and morphometry of circular mounds in the elongated basin of northern Terra Sirenum, Mars, Progress in Earth and Planetary Science, 4, 26, https://doi.org/10.1186/s40645-017-0141-x, 2017.
Hemmi, R. and Miyamoto, H.: High-resolution topographic analyses of mounds in southern Acidalia Planitia, Mars: Implications for possible mud volcanism in submarine and subaerial environments, Geosciences 8, 152, https://doi.org/10.3390/geosciences8050152, 2018.
Hodson, A. J., Nowak, A., Hornum, M. T., Senger, K., Redeker, K., Christiansen, H. H., Jessen, S., Betlem, P., Thornton, S. F., Turchyn, A. V., Olaussen, S., and Marca, A.: Sub-permafrost methane seepage from open-system pingos in Svalbard, The Cryosphere, 14, 3829–3842, https://doi.org/10.5194/tc-14-3829-2020, 2020.
Hon, K., Kauahikaua, J., Denlinger, R., and Mackay, K.: Emplacement and inflation of pahoehoe sheet flows: observation and measurements of active lava flows on Kilauea Volcano, Hawaii, Geol. Soc. Am. Bull., 106, 351–370, https://doi.org/10.1130/0016-7606(1994)106%3C0351:EAIOPS%3E2.3.CO;2, 1994.
Huang, H., Liu, J., Wang, X., Chen, Y., Zhang, Q., Liu, D., Yan,W., and Ren, X.: The Analysis of Cones within the Tianwen-1 Landing Area, Remote Sens.-Basel, 14, 2590, https://doi.org/10.3390/rs14112590, 2022.
Hurst, A., Cartwright, J. A., Huuse, M., and Duranti, D.: Extrusive sandstones (extrudites): a new class of stratigraphic trap?, Geological Society, London, Special Publications, 254, p. 289, 2006.
Inan, S., Yalcin, M. N., Guliyev, I. S., Kuliev, K., and Feyzullayev, A. A.: Deep Petroleum occurrences in the Lower Kura Depression, South Caspian Basin, Azerbaijan: an organic geochemical and basing modeling study, Mar. Petrol. Geol., 14, 731–762, https://doi.org/10.1016/s0264-8172(97)00058-5, 1997.
Ivanov, M. A. and Hiesinger, H.: The Acidalia Mensa region on Mars: A key element to test the Mars ocean hypothesis, Icarus, 349, 113874, https://doi.org/10.1016/j.icarus.2020.113874, 2020.
Ivanov, M. A., Hiesinger, H., Erkeling, G., Hielscher, F., and Reiss, D.: Major episodes of geologic history of Isidis Planitia on Mars, Icarus, 218, 24–46, https://doi.org/10.1016/j.icarus.2011.11.029, 2012.
Ivanov, M. A., Hiesinger, H., Erkeling, G., and Reiss, D.: Mud volcanism and morphology of impact craters in Utopia Planitia on Mars: Evidence for the ancient ocean, Icarus, 228, 121–140, https://doi.org/10.1016/j.icarus.2013.09.018, 2014.
Ivanov, M. A., Hiesinger, H., Erkeling, G., and Reiss, D.: Evidence for large reservoirs of water/mud in Utopia and Acidalia Planitiae on Mars, Icarus, 248, 383–391, https://doi.org/10.1016/j.icarus.2014.11.013, 2015.
Jakosky, B. M.: Atmospheric Loss to Space and the History of Water on Mars, Annu. Rev. Earth Pl. Sc., 49, 71–93, https://doi.org/10.1146/annurev-earth-062420-052845, 2021.
Jakubov, A. A., AliZade, A. A., and Zeinalov, M. M.: Mud volcanoes of the Azerbaijan SSR: Atlas, Azerbaijan Academy of Sciences, Baku, 1971 (in Russian).
Jamtveit, B., Svensen, H., Podladchikov, Y., and Planke, S.: Hydrothermal vent complexes associated with sill intrusions in sedimentary basins, Geological Society, London, Special Publications, 234, 233–241, 2004.
Jolly, R. J. H. and Lonergan, L.: Mechanism and control on the formation of sand intrusion, J. Geol. Soc. London, 159, 5, 605–617, https://doi.org/10.1144/0016-764902-025, 2002.
Jones, E., Caprarelli, G., and Osinski, G. R.: Insights into complex layered ejecta emplacement and subsurface stratigraphy in Chryse Planitia, Mars, through an analysis of THEMIS brightness temperature data, J. Geophys. Res.-Planet., 121, 986–1015. https://doi.org/10.1002/2015JE004879, 2016.
Jöns, H.-P.: Late sedimentation and late sediments in the northern lowlands on Mars, in: Lunar and Planetary Science, XVI, 11–15 March 1985, The Woodlands, TX, 414–415, 1985.
Karagoz, O., Kenkmann, T., and Wulf, G.: Insights into the subsurface structure of wrinkle ridges on Mars, Earth Planet. Sci. Lett., 595, 117759, https://doi.org/10.1016/j.epsl.2022.117759, 2022.
Keszthelyi, L., Jaeger, W., McEwen, A., Tornabene, L., Beyer, R. A., Dundas, C., and Milazzo, M.: High resolution imaging science experiment (HiRISE) images of volcanic terrains from the first 6 months of the Mars reconnaissance orbiter primary science phase, J. Geophys. Res., 113, E04005, https://doi.org/10.1029/2007JE002968, 2008.
Khlystov, O., Poort, J., Mazzini, A., Akhamanov, G. G., Minami, H., Hachikubo, A., Khabuev, A., Kazakov., A. V., De Batist, M., Naudts, L., Chensky, A. G., and Vorobeva, S.: Shallow-rooted mud volcanism in Lake Baikal, Mar. Petrol. Geol., 102, 580–589, https://doi.org/10.1016/j.marpetgeo.2019.01.005, 2019.
Kite, E. S., Hovius, N., Hillier, J. K., and Besserer, J.: Candidate Mud Volcanoes in the Northern Plains of Mars, American Geophysical Union, Fall Meeting 2007, 31st Lunar and Planetary Science, 13–17 March 2007, The Woodlands, TX, abstract id.V13B-1346, 2007.
Kite, E. S., Williams, J.-P., Lucas, A., and Aharonson, O.: Low palaeopressure of the martian atmosphere estimated from the size distribution of ancient craters, Nat. Geosci., 7, 335–339, https://doi.org/10.1038/ngeo2137, 2014.
Knapmeyer, M., Oberst, J., Hauber, E., Wählisch, M., Deuchler, C., and Wagner, R.: Working models for spatial distribution and level of Mars' seismicity, J. Geophys. Res., 111, E11006, https://doi.org/10.1029/2006JE002708, 2006.
Knittel, K. and Boetius, A.: Anaerobic Oxidation of Methane: Progress with an Unknown Process, Annu. Rev. Microbiol., 63, 311–334, https://doi.org/10.1146/annurev.micro.61.080706.093130, 2009.
Knutsen, E. W., Villanueva, G. L., Liuzzi, G., Crismani, M. M. J., Mumma, M. J., Smith, M. D., Vandaele, A. C., Aoki, S., Thomas, I. R., Daerden, F., Viscardy, S., Erwin, J. T., Trompet, L., Neary, L., Ristic, B., Lopez-Valverde, M. A., Lopez-Moreno, J. J., Patel, M. R., Karatekin, O., and Bellucci, G.: Comprehensive investigation of Mars methane and organics with ExoMars/NOMAD, Icarus, 357, 114266, https://doi.org/10.1016/j.icarus.2020.114266, 2021.
Kokoschka, S., Dreier, A., Romoth, K., Taviani, M., Schäfer, N., Reitner, J., and Hoppert, M.: Isolation of Anaerobic Bacteria from Terrestrial Mud Volcanoes (Salse di Nirano, Northern Apennines, Italy), Geomicrobiol. J., 32, 355–364, https://doi.org/10.1080/01490451.2014.940632, 2015.
Komatsu, G.: Rivers in the Solar System: Water is not the only fluid flow on planetary bodies, Geography Compass, 1/3, 480–502, https://doi.org/10.1111/j.1749-8198.2007.00029.x, 2007.
Komatsu, G.: A possible mud volcano field in Chryse Planitia, Mars, in: European Planetary Science Congress, 19–24 September 2010, Rome, Abstract, EPSC2010-131, 2010.
Komatsu, G. and Baker V. R.: Paleohydrology and flood geomorphology of Ares Vallis, J. Geophys. Res., 102, 4151–4160, https://doi.org/10.1029/96JE02564, 1997.
Komatsu, G. and Brož, P.: Southern Chryse Planitia on Mars as a potential landing site: investigation of hypothesized sedimentary volcanism, in: 52th Lunar and Planet. Sci. Conf., 15–19 March 2021, virtual meeting, #1164, 2021.
Komatsu, G. and Ori, G. G.: Exobiological implications of potential sedimentary deposits on Mars, Planet. Space Sci., 48/11, 1043–1052, https://doi.org/10.1016/S0032-0633(00)00078-7, 2000.
Komatsu, G., Ori, G. G., Cardinale, M., Dohm, J. M., Baker, V. R., Vaz, D. A., Ishimaru, R., Namiki, N., and Matsui, T.: Roles of methane and carbon dioxide in geological processes on Mars, Planet. Space Sci., 59, 169–181, https://doi.org/10.1016/j.pss.2010.07.002, 2011.
Komatsu, G., Ishimaru, R., Miyake, N., Ohno, S., and Matsui, T.: Astrobiological Potential of Mud Volcanism on Mars, in: 45th Lunar and Planet. Sci. Conf., 17–21 March 2014, The Woodlands, TX, #1085, 2014.
Komatsu, G., Okubo, C. H., Wray, J. J., Ojha, L., Cardinale, M., Murana, A., Orosei, R., Chan, M. A., Ormö, J., and Gallagher, R.: Small edifice features in Chryse Planitia, Mars: Assessment of a mud volcano hypothesis, Icarus, 268, 56–75, https://doi.org/10.1016/j.icarus.2015.12.032, 2016.
Komatsu, G., Ishimaru, R., Kawai, K., Miyake, N., Kobayashi, M., Sakuma, H., and Matsui, T.: Sedimentary records of ancient mud volcanism: How do we identify mud volcanoes in the stratigraphy of Mars?, in: 50th Lunar and Planet. Sci. Conf., 18–22 March 2019, The Woodlands, TX, #2132, 2019.
Kopf, A. J.: Significance of mud volcanism, Rev. Geophys., 40, 2–52, https://doi.org/10.1029/2000RG000093, 2002.
Korablev, O., Vandaele, A. C., Montmessin, F., Fedorova, A. A., Trokhimovskiy, A., Forget, F., Lefèvre, F., Daerden, F., Thomas, I. R., Trompet, L., Erwin, J. T., Aoki, S., Robert, S., Neary, L., Viscardy, S., Grigoriev, A. V., Ignatiev, N. I., Shakun, A., Patrakeev, A., Belyaev, D. A., Bertaux, J.-L., Olsen, K. S., Baggio, L., Alday, J., Ivanov, Y. S., Ristic, B., Mason, J., Willame, Y., Depiesse, C., Hetey, L., Berkenbosch, S., Clairquin, R., Queirolo, C., Beeckman, B., Neefs, E., Patel, M. R., Bellucci, G., López-Moreno, J.-J., Wilson, C. F., Etiope, G., Zelenyi, L., Svedhem, H., Vago, J. L., and The ACS and NOMAD Science Teams: No detection of methane on Mars from early ExoMars Trace Gas Orbiter observations, Nature, 568, 517–520, https://doi.org/10.1038/s41586-019-1096-4, 2019.
Kossacki, K. J., Markiewicz, W. J., Smith, M. D., Page, D., and Murray., J.: Possible remnants of a frozen mud lake in southern Elysium, Mars, Icarus, 181, 363–374, https://doi.org/10.1016/j.icarus.2005.11.018, 2006.
Krasnopolsky, V. A., Maillard, J. P., and Owen, T. O.: Detection of methane in the martian atmosphere: evidence for life?, Icarus, 172, 537–547, https://doi.org/10.1016/j.icarus.2004.07.004, 2004.
Kreslavsky, M. A. and Head, J. W.: Fate of outflow channel effluents in the northern lowlands of Mars: The Vastitas Borealis Formation as a sublimation residue from frozen ponded bodies of water, J. Geophys. Res.-Planets, 107, 4-1–4-25, https://doi.org/10.1029/2001je001831, 2002.
Kumar, P. S., Krishna, N., Prasanna Lakshmi, K. J., Raghukanth, S. T. G., Dhabu, A., and Platz, T.: Recent seismicity in Valles Marineris, Mars: Insights from young faults, landslides, boulder falls and possible mud volcanoes, Earth Planet. Sc. Lett., 505, 51–64, https://doi.org/10.1016/j.epsl.2018.10.008, 2019.
Lee, D.-H., Kim, J.-H., Lee, Y. M., Kim, J.-H., Jin, Y. K., Paull, C., Ryu, J.-S., and Shin, K.-H.: Geochemical and Microbial Signatures of Siboglinid Tubeworm Habitats at an Active Mud Volcano in the Canadian Beaufort Sea, Front. Mar. Sci., 8, 656171, https://doi.org/10.3389/fmars.2021.656171, 2021.
Lefèvre, F. and Forget F.: Observed variations of methane on Mars unexplained by known atmospheric chemistry and physics, Nature, 460, 720–72, https://doi.org/10.1038/nature08228, 2009.
Li, J., Beghein, C., Lognonné, P., McLennan, S. M., Wieczorek, M. A., Panning, M. A., Knapmeyer-Endrun, B., Davis, P., and Banerdt, W. B.: Different Martian Crustal Seismic Velocities across the Dichotomy Boundary from Multi-Orbiting Surface Waves, Geophys. Res. Lett., 49, e2022GL101243, https://doi.org/10.1029/2022GL101243, 2022.
Lin, Y., Zhao, J., Wang, L., Huang, J., Zhang, L., and Xiao, L.: Evaluation of small-sized mounds formation mechanisms in China's Zhurong landing region, Icarus, 389, 115256, https://doi.org/10.1016/j.icarus.2022.115256, 2023.
Liu, J., Li, C., Zhang, R., Rao, W., Cui, X., Geng, Y., Jia, Y., Huang, H., Ren, X., Yan, W., Zeng, X., Wen, W., Wang, X., Gao, X., Fu, Q., Zhu, Y., Dong, J., Li, H., Wang, X., Zuo, W., Su, Y., Kong, D., and Zhang, H.: Geomorphic Contexts and Science Focus of the Zhurong Landing Site on Mars, Nat. Astron., 6, 65–71, https://doi.org/10.1038/s41550-021-01519-5, 2021.
Lognonné, P., Banerdt, W. B., Clinton, J., Garcia, R.F, Giardini, D., Knapmeyer-Endrun, B., Panning, M., and Pike, W. T.: Mars Seismology, Annu. Rev. Earth Pl. Sc., 51, 643–670, https://doi.org/10.1146/annurev-earth-031621-073318, 2023.
Lucchitta, B. K.: Young volcanic deposits in the Valles Marineris, Mars?, Icarus, 86, 476–509, https://doi.org/10.1016/0019-1035(90)90230-7, 1990.
Lucchitta, B. K., Ferguson, H. M., and Summers, C.: Sedimentary deposits in the Northern Lowland Plains, Mars, J. Geophys. Res., 91, E166–E174, https://doi.org/10.1029/JB091iB13p0E166, 1986.
Mahaney, W. C., Milner, M. W., Netoff, D. I., Malloch, D., Dohm, J. M., Baker, V. R., Miyamoto, H., Hare, T. M., and Komatsu, G.: Ancient wet aeolian environments on Earth: clues to presence of fossil/live microorganisms on Mars, Icarus, 171, 39–53, 2004.
Malin, M. C. and Edgett, K. S.: Sedimentary rocks of early Mars, Science, 290, 1927–1937, https://doi.org/10.1126/science.290.5498.1927, 2000.
Mazzini, A. and Etiope, G.: Mud volcanism: an updated review, Earth Sci. Rev., 168, 81–112, https://doi.org/10.1016/j.earscirev.2017.03.001, 2017.
Mazzini, A., Svensen, H., Planke, S., Guliyev, I., Akhmanov, G. G., Fallik, T., and Banks, D.: When mud volcanoes sleep: Insight from seep geochemistry at the Dashgil mud volcano, Azerbaijan, Mar. Petrol. Geol., 26, 1704–1715, 2009.
Mazzini A., Etiope, G., and Svensen H.: A new hydrothermal scenario for the 2006 Lusi eruption, Indonesia. Insights from gas geochemistry, Earth Planet. Sc. Lett., 317–318, 305–318, 2012.
Mazzini, A., Akhmanov, G., Manga, M., Sciarra, A., Huseynova, A., Huseynov, A., and Guliyev, I.: Explosive mud volcano eruptions and rafting of mud breccia blocks, Earth Planet. Sc. Lett., 555, 116699, https://doi.org/10.1016/j.epsl.2020.116699, 2021.
McCauley, C. A., White, D. M., Lilly, M. R., and Nyman, D. M.: A comparison of hydraulic conductivities, permeabilities and infiltration rates in frozen and unfrozen soils, Cold Reg. Sci. Technol., 34, 117–125, https://doi.org/10.1016/S0165-232X(01)00064-7, 2002.
McGill, G. E.: Buried topography of Utopia, Mars: Persistence of a giant impact depression, J. Geophys. Res.-Sol. Ea., 94, 5622935, https://doi.org/10.1029/JB094iB03p02753, 1989.
McGowan, E.: Spatial distribution of putative water related features in Southern Acidalia/Cydonia Mensae, Mars, Icarus, 202, 78–89, 2009.
McGowan, E. M. and McGill, G. E.: The Utopia/Isidis overlap; possible conduit for mud volcanism, in: 41st Lunar and Planetary Science Conference, 1–5 March 2010, The Woodlands, TX, Abs. # 1070, 2010.
McNeil, J. D., Fawdon, P., Balme, M. R., and Coe, A. L.: Morphology, morphometry and distribution of isolated landforms in southern Chryse Planitia, Mars, J. Geophys. Res.-Planet., 126, e2020JE006775, https://doi.org/10.1029/2020JE006775, 2021.
Meresse, S., Costard, F.,Mangold, N., Masson, P., Neukum, G., and the HRSC Co-I Team: Formation and evolution of the chaotic terrains by subsidence and magmatism: Hydraotes Chaos, Mars, Icarus, 194, 487–500. https://doi.org/10.1016/j.icarus.2007.10.023, 2008.
Miall, A. D.: Principles of Sedimentary Basin Analysis, Springer, New York, https://doi.org/10.1007/978-1-4757-4235-0, 1984.
Michalski, J. R., Cuadros, J., Niles, P. B., Parnell, J., Rogers, A. D., and Wright, S. P.: Groundwater activity on Mars and implications for a deep biosphere, Nat. Geosci., 6, 133–138, https://doi.org/10.1038/ngeo1706, 2013.
Milkov, A. V., Sassen, R., Apanasovich, T. V., and Dadashev, F. G.: Global gas flux from mud volcanoes: A significant source of fossil methane in the atmosphere and the ocean, Geophys. Res. Lett., 30, 1037, https://doi.org/10.1029/2002GL016358, 2003.
Milliken, R. E., Grotzinger, J. P., and Thomson, B. J.: Paleoclimate of Mars as captured by the stratigraphic record in Gale Crater, Geophys. Res. Lett., 37, L04201, https://doi.org/10.1029/2009GL041870, 2010.
Miyake, N., Ishimaru, R., Komatsu, G., and Matsui, T.: Characterization of archaeal and bacterial communities thriving in methane-seeping on-land mud volcanoes, Niigata, Japan, Int. Microbiol., 26, 191–204, https://doi.org/10.1007/s10123-022-00288-z, 2023.
Möhlmann, D. T. F.: Water in the upper martian surface at mid- and low-latitudes: presence, state, and consequences, Icarus, 168, 318–323, https://doi.org/10.1016/j.icarus.2003.11.008, 2004.
Moscardelli, L., Dooley, T., Dunlap, D., Jackson, M., and Wood, L.: Deep-water polygonal fault systems as terrestrial analogs for large-scale Martian polygonal terrains, GSA Today, 22, 4–9, https://doi.org/10.1130/GSATG147A.1, 2012.
Mouginis-Mark, P. J.: A unique young flow southwest of Cerberus Fossae, Mars, in: 44th Lunar and Planetary Science Conference, 18–22 March 2013, The Woodlands, TX, abstract #1203, 2013.
Mueller, K. and Golombek, M.: Compressional Structures on Mars. Annu. Rev. Earth Pl. Sc., 32, 435–464, https://doi.org/10.1146/annurev.earth.32.101802.120553, 2004.
Mumma, M. J., Villanueva, G. L., Novak, R. E., Hewagama, T., Bonev, B. P., DiSanti, M. A., Mandell, A. M., and Smith, M. D.: Strong Release of Methane on Mars in Northern Summer 2003, Science, 323, 1041–1045, https://doi.org/10.1126/science.1165243, 2009.
Mustard, J. F., Ehlmann, B. L., Murchie, S. L., Poulet, F., Mangold, N., Head, J. W., Bibring, J.-P., and Roach, L. H.: Composition, morphology and stratigraphy of Noachian crust around the Isidis Basin, J. Geophys. Res., 114, E00D12, https://doi.org/10.1029/2009JE003349, 2009.
Oberst, J., Wickhusen, K., Gwinner, K., Hauber, E., Stark, A., Elgner, S., Grott, M., Fanara, L., Hussmann, H., Steinbrügge, G., Lewis, S., Balme, M., Maugeri, M., Diolaiuti, G., Karlsson, N., Johnsson, A., Ivanov, A., and Hiesinger, H.: Planetary polar explorer – the case for a next-generation remote sensing mission to low Mars orbit, Exp. Astron., 54, 695–711, https://doi.org/10.1007/s10686-021-09820-x, 2022.
Oehler, D. and Etiope G.: Methane Seepage on Mars: Where to Look and Why, Astrobiology, 17, 1233–1264, https://doi.org/10.1089/ast.2017.1657, 2017.
Oehler, D. and Etiope G.: Methane on Mars: subsurface sourcing and conflicting atmospheric measurements, in: Mars Geological Enigmas: From the Late Noachian Epoch to the Present Day, 1st edn., edited by: Soare, R., Conway, S., Williams, J. P., and Oehler D., Elsevier, 149–174, https://doi.org/10.1016/b978-0-12-820245-6.00007-0, 2021.
Oehler, D. Z. and Allen, C. C.: Mud volcanoes in the martian lowlands: potential windows to fluid-rich samples from depth, 40th Lunar and Planetary Science Conference, 23–27 March 2009, The Woodlands, TX, Abs. # 1034, 2009.
Oehler, D. Z. and Allen, C. C.: Evidence for pervasive mud volcanism in Acidalia Planitia, Mars, Icarus, 208, 636–657, 2010.
Oehler, D. Z. and Allen, C. C.: Habitability of a large ghost crater in Chryse Planitia, Mars, in: International Conference: Exploring Mars Habitability, 13–15 June 2011, Lisbon, Portugal, https://sci.esa.int/documents/33431/35950/1567258599260-Oehler.pdf (last access: 17 July 2023), 2011.
Oehler, D. Z. and Allen, C. C.: Giant polygons and mounds in the lowlands of Mars: Signatures of an ancient ocean?, Astrobiology, 12, 601–615, https://doi.org/10.1089/ast.2011.0803, 2012a.
Oehler, D. Z. and Allen, C. C.: Focusing the search for biosignatures on Mars: Facies prediction with an example from Acidalia Planitia, in: Sedimentary Geology of Mars, SEPM Special Publ., No. 102, Society for Sedimentary Geology, 183–194, https://doi.org/10.2110/pec.12.102.0183, 2012b.
Oehler, D. Z., Salvatore, M., Etiope, G., and Allen. C. C.: Focusing the search for organic biosignatures on Mars, 52nd Lunar and Planetary Science Conference, 15–19 March 2021, The Woodlands, TX, Abs. # 1353, 2021.
Okubo, C. H.: Morphologic evidence of subsurface sediment mobilization and mud volcanism in Candor and Coprates Chasmata, Valles Marineris, Mars, Icarus, 269, 23–37, https://doi.org/10.1016/j.icarus.2015.12.051, 2016.
Orgel, C., Hauber, E., Skinner Jr., J. A., van Gasselt, S., Ransdale, J., Balme, M., Séjourné, A., and Keresz-Turi, A.: Distribution, origin and evolution of hypothesized mud volcanoes, thumbprint terrain and giant polygons in Acidalia, Utopia and Arcadia Planitae: Implications for sedimentary processes in the northern lowlands of Mars, in: 46th Lunar and Planetary Science Conference, 16–20 March 2015, The Woodlands, TX, Abs. 1862, 2015.
Orgel, C., Hauber, E., Van Gasselt, S., Reiss, D., Johnsson, A., Ramsdale, J. D., Smith, I., Swirad, Z. M., Séjourné, A., Wilson, J. T., Balme, M. R., Conway, S. J., Costard, F., Eke, V. R., Gallagher, C., Kereszturi, A., Losiak, A., Massey, R. J., Platz, T., Skinner, J. A., and Teodoro, L. R. F.: Grid mapping the northern plains of Mars: A new overview of recent water- and ice-related landforms in Acidalia Planitia, J. Geophys. Res.-Planet., 124, 454–482, https://doi.org/10.1029/2018JE005664, 2019.
Ori, G. G., Marinangeli, L., and Komatsu, G.: Gas (methane?)-related features on the surface of Mars and subsurface reservoirs, in: Proceedings of the Lunar Planetary Science Conference XXXI, 13–17 March 2000, Houston, TX, #1550, abstract [CD-ROM], 2000.
Ori, G. G., Komatsu, G., Ormö, J., and Marinangeli, L.: Subsurface models for the formation of mound-like morphologies on Mars, in: Proceedings of the Lunar Planetary Science Conference XXXII, 12–16 March 2001, Houston, TX, #1539, abstract [CD-ROM], 2001.
Ormö, J., Komatsu, G., Chan, M. A., Beitler, B., and Parry, W. T.: Geological features indicative of processes related to the hematite formation in Meridiani Planum and Aram Chaos, Mars: A comparison with diagenetic hematite deposits in southern Utah, USA, Icarus, 171, 295–316, https://doi.org/10.1016/j.icarus.2004.06.001, 2004.
Pajola, M., Rossato, S., Baratti, E., Mangili, C., Mancarella, F., McBride, K., and Coradini, M.: The Simud–Tiu Valles hydrologic system: A multidisciplinary study of a possible site for future Mars on-site exploration, Icarus, 268, 355–381, https://doi.org/10.1016/j.icarus.2015.12.049, 2016.
Pan, L., Ehlmann, B. L., Carter, J., and Ernst, C. M.: The stratigraphy and history of Mars' northern lowlands through mineralogy of impact craters: A comprehensive survey, J. Geophys. Res.-Planet., 122, 1824–1854, https://doi.org/10.1002/2017JE005276, 2017.
Parfitt, E. A. and Wilson, L.: Fundamentals of Physical Volcanology, Blackwell, Oxford, UK, 256 pp., ISBN 978-0-632-05443-5, 2008.
Pavlov, A. A., McLain, H. L., Glavin, D. P., Roussel, A.,Dworkin, J. P., Elsila, J. E., and Yocum, K. M.: Rapid Radiolytic Degradation of Amino Acids in the Martian Shallow Subsurface: Implications for the Search for Extinct Life, Astrobiology, 22, 1099–1115, https://doi.org/10.1089/ast.2021.0166, 2022.
Pfeffer, W. and Humphrey, N.: Formation of ice layers by infiltration and refreezing of meltwater, Ann. Glaciol., 26, 83–91, https://doi.org/10.3189/1998AoG26-1-83-91, 1998.
Plümper, O., King, H. E., Geisler, T., Liu, Y., Pabst, S., Savov, I. P., Rost, D., and Zack, T.: Subduction zone forearc serpentinites as incubators for deep microbial life, P. Natl. Acad. Sci. USA, 114, 4324–4329, https://doi.org/10.1073/pnas.1612147114, 2017.
Polteau, S., Mazzini, A., Galland, O., Planke, S., and Malthe-Sorenssen, A.: Saucer-shaped intrusions: Occurrences, emplacement and implications, Earth Planet. Sc. Lett., 266, 195–204, https://doi.org/10.1016/j.epsl.2007.11.015, 2008.
Pondrelli, M., Rossi, A. P., Ori, G. G., Van Gasselt, S., Praeg, D., and Ceramicola, S.: Mud volcanoes in the geologic record of Mars: the case of Firsoff Crater, Earth Planet. Sc. Lett., 304, 511–519, https://doi.org/10.1016/j.epsl.2011.02.027, 2011.
Pons, M.-L., Quitté, G., Fujii, T., Rosing, M. T., Reynard, B., Moynier, F., Douchet, C., and Albarède, F.: Early Archean serpentine mud volcanoes at Isua, Greenland, as a niche for early life, P. Natl. Acad. Sci. USA, 108, 17639–17643, https://doi.org/10.1073/pnas.1108061108, 2011.
Procesi, M., Ciotoli, G., Mazzini, A., and Etiope, G.: Sediment-Hosted Geothermal Systems: review and first global mapping, Earth Sci. Rev., 192, 529–544, https://doi.org/10.1016/j.earscirev.2019.03.020, 2019.
Quantin, C., Flahut, J., Clenet, H., Allemand, P., and Thomas, P.: Composition and structures of the subsurface in the vicinity of Valles Marineris as revealed by central uplifts of impact craters, Icarus, 221, 436–452, https://doi.org/10.1016/j.icarus.2012.07.031, 2012.
Rice Jr., J. W., and Edgett, K. S.: Catastrophic flood sediments in Chryse basin, Mars, and Quincy basin, Washington: application of sandar facies model, J. Geophys. Res., 102, 4185–4200, https://doi.org/10.1029/96JE02824, 1997.
Rodríguez, J. A. P., Tanaka, K. L., Kargel, J. S., Dohm, J. M., Kuzmin, R., Fairén, A. G., Sasaki, S., Komatsu, G., Schulze-Makuch, D., and Jianguo, Y.: Formation and disruption of aquifers in southwestern Chryse Planitia, Mars, Icarus, 191, 545–567, https://doi.org/10.1016/j.icarus.2007.05.021, 2007.
Rodríguez, J. A. P., Fairén, A.G., Tanaka, K. L., Zarroca, M., Linares, R., Platz, T., Komatsu, G., Miyamoto, H., Kargel, J. S., Yan, J., Gulick, V., Higuchi, K., Baker, V. R., and Glines, N.: Tsunami waves extensively resurfaced the shorelines of a receding, early Martian ocean, Sci. Rep.-UK, 6, 25106, https://doi.org/10.1038/srep25106, 2016.
Rodríguez, J. A. P., Kargel, J. S., Oehler, D. Z., Crown, D. A., Baker, V. R., and Komatsu, G.: Potential cryospheric mud volcanism in the northern plains of Mars, Geologic and astrobiological implications, in: 50th Lunar and Planetary Science Conference, 18–22 March 2019, The Woodlands, TX, Abs. # 2580, 2019.
Rowland, S. K., Harris, A., L., and Garbeil, H.: Effects of Martian conditions on numerically modeled, cooling-limited, channelized lava flows, J. Geophys. Res.-Planet., 109, Issue E10, https://doi.org/10.1029/2004JE002288, 2004.
Rubin, D. M., Faíren, A., Martínez-Frías, J., Frydenvang, J., Gasnault, O., Galfenbaum, G., Goetz, W., Grotzinger, J. P., Le Mouélic, S., Mangold, N., Newsom, H., Oehler, D. Z., Rapin, W., Schieber, J., and Weins, R. C.: Fluidized-sediment pipes in Gale Crater, Mars, and possible Earth analogs, Geology 45, 7–10, https://doi.org/10.1130/G38339.1, 2016.
Salvatore, M. R. and Christensen, P. R.: Evidence for widespread aqueous sedimentation in the northern plains of Mars, Geology 42, 423–426, https://doi.org/10.1130/G35319.1, 2014a.
Salvatore, M. R. and Christensen, P. R.: On the origin of the Vastitas Borealis Formation in Chryse and Acidalia Planitiae, Mars, J. Geophys. Res.-Planet., 119, 2437–2456, https://doi.org/10.1002/2014JE004682, 2014b.
Scott, D. H. and Tanaka, K. L.: Geologic map of the western equatorial region of Mars, U.S. Geological Survey Miscellaneous Investigations Series Map I-1802, Scale , U.S. Geological Survey, https://doi.org/10.3133/i1802A, 1986.
Scott, D. H. and Underwood Jr., J. R.: Mottled terrain – A continuing martian enigma, in: 21st Lunar and Planetary Science Conference, 12–16 March 1991, The Woodlands, TX, 627–634, 1991.
Skinner Jr., J. A. and Mazzini, A.: Martian mud volcanism: terrestrial analogs and implications for formational scenarios, Mari. Petrol. Geol., 26, 1866–1878, https://doi.org/10.1016/j.marpetgeo.2009.02.006, 2009.
Skinner Jr., J. A. and Tanaka, K. L.: Evidence for and implications of sedimentary diapirism and mud volcanism in the southern Utopia highland–lowland boundary plain, Mars, Icarus, 186, 41–59, https://doi.org/10.1016/j.icarus.2006.08.013, 2007.
Smrekar, S. E., Lognonné, P., Spohn, T., Banerdt, W. B., Breuer, D., Christensen, U., Dehant, V., Drilleau, M., Folkner, W., Fuji, N., Garcia, R.F., Giardini, D., Golombek, M., Grott, M., Gudkova, T., Johnson, C., Khan, A., Langlais, B., Mittelholz, A., Mocquet, A., Myhill, R., Panning, M., Perrin, C., Pike, T., Plesa, A-C., Rivoldini, A., Samuel, H., Stähler, S. C., van Driel, M., Van Hoolst, T., Verhoeven, O., Weber, R., and Wieczorek, M.: Pre-mission InSights on the Interior of Mars, Space Sci. Rev., 215, 3, https://doi.org/10.1007/s11214-018-0563-9, 2019.
Solomon, S. C.: On volcanism and thermal tectonics on one-plate planets, Geophys. Res. Lett., 5, 461-464, https://doi.org/10.1029/GL005i006p00461, 1978.
Stamenković, V., and 98 co-authors: Deep Trek: Science of Subsurface Habitability & Life on Mars, Bulletin of the AAS, 53, 250, https://doi.org/10.3847/25c2cfeb.dc18f731, 2021.
Svensen, H., Jamtveit, B., Planke, S., and Chevallier, L.: Structure and evolution of hydrothermal vent complexes in the Karoo Basin, South Africa, J. Geol. Soc. London, 163, https://doi.org/10.1144/1144-764905-037, 671–682, 2006.
Tanaka, K. L.: Sedimentary history and mass flow structures of Chryse and Acidalia Planitiae, Mars, J. Geophys. Res., 102, 4131–4150, https://doi.org/10.1029/96JE02862, 1997.
Tanaka, K. L.: Debris-flow origin for the Simud/Tiu deposit on Mars, J. Geophys. Res.-Planet., 104, 8637–8652, https://doi.org/10.1029/98JE02552, 1999.
Tanaka, K. L.: Geology and insolation-driven climatic history of Amazonian north polar materials on Mars, Nature, 437, 991–994, https://doi.org/10.1038/nature04065, 2005.
Tanaka, K. L., Joyal, T., and Wenker, A.: The Isidis Plains Unit, Mars: Possible catastrophic origin, tectonic tilting, and sediment loading, in: 31st Lunar and Planetary Science Conference, 13–17 March 2000, The Woodlands, TX, Abs. # 2023. 2000.
Tanaka, K. L., Skinner Jr., J. A., Hare, T. M., Joyal, T., and Wenker, A.: Resurfacing history of the Northern Plains of Mars based on geologic mapping of Mars Global Surveyor data, J. Geophys. Res., 108, GDS 24-1–GDS 24-32, https://doi.org/10.1029/2002JE001908, 2003.
Tanaka, K. L., Skinner Jr., J. A., and Hare, T. M.: Geologic Map of the Northern Plains of Mars, U.S. Geological Survey Science Investigations Map 2888, scale , U.S. Geological Survey, https://pubs.usgs.gov/sim/2005/2888 (last access: 14 July 2023), 2005.
Tanaka, K. L., Rodríguez, J. A. P., Skinner Jr., J. A., Mourke, M. C., Fortezzo, C. M., Herkenhoff, K. E., Kolb, E. J., and Okubo, C. H.: North polar region of Mars: Advances in stratigraphy, structure, and erosional modification, Icarus, 196, 318–358, https://doi.org/10.1016/j.icarus.2008.01.021, 2008.
Tanaka, K. L., Skinner Jr., J. A., Dohm, J. M., Irwin III, R. P., Kolb, E. J., Fortezzo, C. M., Platz, T., Michael, G. G., and Hare T. M.: Geologic Map of Mars, U.S. Geological Survey Scientific Investigations Map 3292, scale , U.S. Geological Survey,, https://doi.org/10.3133/sim3292, 2014.
Tewelde, Y. and Zuber, M. T.: Determining the fill thickness and densities of Mars' northern lowlands, in: 44th Lunar and Planetary Science Conference, 18–22 March 2013, The Woodlands, TX, Abs. #2151, 2013.
Tosi, N. and Padovan, S.: Mercury, Moon, Mars, in: Mantle Convection and Surface Expressions, edited by: Marquardt, H., Ballmer, M., Cottaar, S., and Konter, J., Wiley, https://doi.org/10.1002/9781119528609.ch17, 2021.
Vago, J. L., Westall, F., Coates, A. J., Jaumann, R., Korablev, O., Ciarletti, V., Mitrofanov, I., Josset, J. L., De Sanctis, M. C., Bibring, J. P., Pasteur Teams, and Landing Site Selection Working Group: Habitability on Early Mars and the Search for Biosignatures with the Exomars Rover, Astrobiology, 17, 471–510, https://doi.org/10.1089/ast.2016.1533, 2017.
van Rensbergen, P., Hillis, R. R., Maltman, A. J., and Morley, C. K.: Subsurface sediment mobilization: Introduction, Geological Society, London, Special Publications, 216, 1–8, https://doi.org/10.1144/GSL.SP.2003.216.01.01, 2003.
Wallace, D. and Sagan, C.: Evaporation of ice in planetary atmospheres: ice-covered rivers on Mars, Icarus, 39, 385–400, https://doi.org/10.1016/0019-1035(79)90148-9, 1979.
Warner, N. H., Sowe, M., Gupta, S., Dumke, A., and Goddard, K.: Fill and spill of giant lakes in the eastern Valles Marineris region of Mars, Geology, 41, 675–678, https://doi.org/10.1130/G34172.1, 2013.
Warner, N. H., Gupta, S., Calef, F. Grindrod, P., Boll, N., and Goddard, K.: Minimum effective area for high resolution crater counting of martian terrains, Icarus, 245, 198–240, https://doi.org/10.1016/j.icarus.2014.09.024, 2015.
Webster, C. R., Mahaffy, P. R., Atreya, S. K., Moores, J. E., Flesch, G. J., Malespin, C., McKay, C. P., Martinez, G., Smith, C. L., Martin-Torres, J., Gomez-Elvira, J., Zorzano, M. P., Wong, M. H., Trainer, M. G., Steele, A., Archer, D. Jr., Sutter, B., Coll, P. J., Freissinet, C., Meslin, P. Y., Gough, R. V., House, C. H., Pavlov, A., Eigenbrode, J. L., Glavin, D. P., Pearson, J. C., Keymeulen, D., Christensen, L. E., Schwenzer, S. P., Navarro-Gonzalez, R., Pla-García, J., Rafkin, S. C. R., Vicente-Retortillo, Á., Kahanpää, H., Viudez-Moreiras, D., Smith, M. D., Harri, A. M., Genzer, M., Hassler, D. M., Lemmon, M., Crisp, J., Sander, S. P., Zurek, R. W., and Vasavada, A. R.: Background levels of methane in Mars' atmosphere show strong seasonal variations, Science, 360, 1093–1096, https://doi.org/10.1126/science.aaq0131, 2018.
Werner, S. C., Ody, A., and Poulet, F.: The Source Crater of Martian Shergottite Meteorites, Science, 343, 1343–1346, https://doi.org/10.1126/science.1247282, 2014.
Westall, F., Foucher, F., Bost, N., Bertrand, M., Loizeau, D., Vago, J. L., Kminek, G., Gaboyer, F., Campbell, K. A., Bréhéret, J. G., Gautret, P., and Cockell, CS.: Biosignatures on Mars: What, Where, and How? Implications for the Search for Martian Life, Astrobiology, 11, 998–1029, https://doi.org/10.1089/ast.2015.1374, 2015.
Wheatley, D. F., Chan, M. A., and Sprinkel, D. A.: Clastic pipe characteristics and distributions throughout the Colorado Plateau: Implications for paleoenvironment and paleoseismic controls, Sediment. Geol., 344, 20–33, https://doi.org/10.1016/j.sedgeo.2016.03.027, 2016.
Wheatley, D. F., Chan, M. A., and Okubo, C. H.: Clastic pipes and mud volcanism across Mars: Terrestrial analog evidence of past martian groundwater and subsurface fluid mobilization, Icarus, 328, 141–151, https://doi.org/10.1016/j.icarus.2019.02.002, 2019.
Wieczorek, M. A., Broquet, A., McLennan, S. M., Rivoldini, A., Golombek, M., Antonangeli, D., Beghein, C., Giardini, D., Gudkova, T., Gyalay, S., and Johnson, C. L.: InSight constraints on the global character of the Martian crust, J. Geophys. Res.-Planet., 127, e2022JE007298, https://doi.org/10.1029/2022JE007298, 2022.
Wilson, L. and Head, J. W.: Review and analysis of volcanic eruption theory and relationships to observed landforms, Rev. Geophys., 32, 221–263, https://doi.org/10.1029/94RG01113, 1994.
Wilson, L. and Head, J. W.: Evidence for a massive phreatomagmatic eruption in the initial stages of formation of the Mangala Valles outflow channel, Mars, Geophys. Res. Lett., 31, L15701, https://doi.org/10.1029/2004GL020322, 2004.
Wilson, L. and Mouginis-Mark, P. J.: Dynamics of a fluid flow on Mars: Lava or mud?, Icarus, 233, 268–280, https://doi.org/ {10.1016/j.icarus.2014.01.041}, 2014.
Wordsworth, R. D.: The Climate of Early Mars. Annu. Rev. Earth Pl. Sc., 44, 381–408, https://doi.org/10.1146/annurev-earth-060115-012355, 2016.
Wray, J. J.: Contemporary Liquid Water on Mars? Annu. Rev. Earth Pl. Sc., 49, 141–171, https://doi.org/10.1146/annurev-earth-072420-071823, 2021.
Wrede, C., Brady, S., Rockstroh, S., Dreier, A., Kokoschka, S., Heinzelmann, S. M., and Heller, C.: Aerobic and anaerobic methane oxidation in terrestrial mud volcanoes in the Northern Apennines, Sediment. Geol., 263–264, 210–219, 2012.
Ye, B., Qian, Y., Xiao, L., Michalski, J. R., Li, Y., Wu, B., and Qiao, L.: Geomorphologic exploration targets at the Zhurong landing site in the southern Utopia Planitia of Mars, Earth Planet. Sc. Lett., 576, 117199, https://doi.org/10.1016/j.epsl.2021.117199, 2021.
Zhao, J., Xiao, Z., Huang, J., Head, J. W., Wang, J., Shi, Y., Wu, B., and Wang, L.: Geological characteristics and targets of high scientific interest in the Zhurong landing region on Mars, Geophys. Res. Lett., 48, e2021GL094903, https://doi.org/10.1029/2021GL094903, 2021.
- Abstract
- Introduction
- Surface mud-fluid manifestations on Earth: definitions, origin, and variants
- Observations
- Ideal prerequisites for forming sedimentary volcanism on Mars?
- Addressing the key questions about sedimentary volcanism for Mars
- Ground truthing and tests of the sedimentary volcano hypothesis
- Conclusions
- Data availability
- Author contributions
- Competing interests
- Disclaimer
- Special issue statement
- Acknowledgements
- Financial support
- Review statement
- References
- Supplement
- Abstract
- Introduction
- Surface mud-fluid manifestations on Earth: definitions, origin, and variants
- Observations
- Ideal prerequisites for forming sedimentary volcanism on Mars?
- Addressing the key questions about sedimentary volcanism for Mars
- Ground truthing and tests of the sedimentary volcano hypothesis
- Conclusions
- Data availability
- Author contributions
- Competing interests
- Disclaimer
- Special issue statement
- Acknowledgements
- Financial support
- Review statement
- References
- Supplement