the Creative Commons Attribution 4.0 License.
the Creative Commons Attribution 4.0 License.
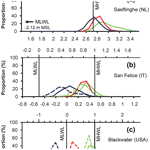
Different coastal marsh sites reflect similar topographic conditions under which bare patches and vegetation recovery occur
Chen Wang
Lennert Schepers
Matthew L. Kirwan
Enrica Belluco
Andrea D'Alpaos
Qiao Wang
Shoujing Yin
Stijn Temmerman
The presence of bare patches within otherwise vegetated coastal marshes is sometimes considered to be a symptom of marsh dieback and the subsequent loss of important ecosystem services. Here we studied the topographical conditions determining the presence and revegetation of bare patches in three marsh sites with contrasting tidal range, sediment supply, and plant species: the Scheldt estuary (the Netherlands), Venice lagoon (Italy), and Blackwater marshes (Maryland, USA). Based on GIS (geographic information system) analyses of aerial photos and lidar imagery of high resolution ( m pixels), we analyzed the topographic conditions under which bare patches occur, including their surface elevation, size, distance from channels, and whether they are connected or not to channels. Our results demonstrate that, for the different marsh sites, bare patches can be connected or unconnected to the channel network and that there is a positive relationship between the width of the connecting channels and the size of the bare patches, in each of the three marsh sites. Further, pixels located in bare patches connected to channels occur most frequently at the lowest elevations and farthest distance from the channels. Pixels in bare patches disconnected from channels occur most frequently at intermediate elevations and distances from channels, and vegetated marshes dominate at highest elevations and shortest distances from channels. In line with previous studies, revegetation in bare patches is observed in only one site with the highest tidal range and highest sediment availability, and it preferentially occurs from the edges of small unconnected bare patches at intermediate elevations and intermediate distances from channels. Although our study is only for three different marsh sites with large variations in local conditions, such as tidal range, sediment availability, and plant species, it suggests that similar topographic conditions determine the occurrence of bare patches. Such insights may inform decision makers on coastal marsh management on where to focus monitoring of early signatures of marsh degradation.
- Article
(7860 KB) - Full-text XML
-
Supplement
(1425 KB) - BibTeX
- EndNote
Tidal marshes are coastal ecosystems that provide many valuable ecosystem services such as fishery production (Barbier et al., 2011), sequestration of CO2 (McLeod et al., 2011), protection against shoreline erosion, and mitigation of flood risks during storm surges (Barbier et al., 2008; Wamsley et al., 2010; Gedan et al., 2011; Temmerman et al., 2013; Temmerman and Kirwan, 2015). However, tidal marshes and their valuable ecosystem services can be lost when marshes die-off, for instance, as a consequence of sea level rise. Large-scale tidal marsh loss by conversion of marshes into bare tidal flats, open water, or bare patches within marshes has been reported from different locations around the world (Baumann et al., 1984; Day et al., 2000; Kearney et al., 2002; Carniello et al., 2009; Kirwan and Megonigal, 2013). Bare patches within marshes, which are often covered by standing water and then referred to as pools, ponds (Stevenson et al., 1985), or marsh basins (Mariotti and Fagherazzi, 2013), are a common feature in salt marshes around the world. In some regions, bare patches are dynamic features that develop but also recover and revegetate (e.g., New England; Wilson et al., 2009, 2010, 2014). In other areas, however, bare patches do not revegetate and are causing permanent marsh loss on a large scale (e.g., Mississippi Delta; Penland et al., 2000; Morton et al., 2003).
Marsh loss and recovery are of particular concern because there is growing evidence that vegetated marshes and bare flats behave as alternative stable ecosystem states (Fagherazzi et al., 2006; Kirwan and Murray, 2007; Marani et al., 2007, 2010; van Wesenbeeck et al., 2008; D'Alpaos, 2011; McGlathery et al., 2013; Wang and Temmerman, 2013; Moffett et al., 2015; D'Alpaos and Marani, 2016; van Belzen et al., 2017), which implies that recovery after marsh loss would be very difficult (Hu et al., 2015a; van Belzen et al., 2017). Observations have shown that vegetated marshes and bare flats occupy different elevation ranges (Marani et al., 2007, 2010; Carniello et al., 2009; Wang and Temmerman, 2013) and that shifts from the low-lying bare state to the high-elevation vegetated state occur rapidly once a threshold elevation has been exceeded (Wang and Temmerman, 2013). Moreover, models indicate that the system would shift abruptly between the high-elevation vegetated state and low-lying bare state when a threshold value is reached in elevation, sediment input, or rate of sea level rise (Fagherazzi et al., 2007; Kirwan and Guntenspergen, 2010; Marani et al., 2010; D'Alpaos et al., 2011; D'Alpaos and Marani, 2016). Previous studies further suggest that the state shift can be irreversible because of a hysteresis effect (Kirwan and Murray, 2007; Marani et al., 2010; Kirwan et al., 2011), where the threshold conditions to revert the ecosystem back to the original state are far more difficult to reach than the threshold conditions that caused the shift (Scheffer et al., 2001; Scheffer and Carpenter, 2003). Field experiments have also demonstrated that vegetation recovery after disturbance is slower under increased tidal inundation, which further suggests the applicability of alternative stable state theory to vegetated and bare areas in intertidal zones (van Belzen et al., 2017).
The two stable states of marshes and tidal flats can be explained by positive feedback mechanisms which are strongly mediated by the presence or absence of marsh vegetation. As long as vegetation is present on the marsh, waves and tidal currents are effectively attenuated by vegetation-induced friction over several meters of continuously vegetated marsh surfaces (Neumeier and Amos, 2006; Mudd et al., 2010; Vandenbruwaene et al., 2011; Yang et al., 2012; Hu et al., 2014). As a consequence, suspended sediment is deposited on the marsh surface and marshes can maintain a high position in the tidal frame, even with sea level rise (Kirwan and Guntenspergen, 2010; D'Alpaos et al., 2011; Fagherazzi et al., 2012). Above- and belowground plant material further contributes to marsh accretion (Nyman et al., 2006; Kirwan and Guntenspergen, 2012). When vegetation is absent, however, organic matter accumulation is strongly reduced, and increased tidal currents and waves may prevent sedimentation or even trigger erosion (Fagherazzi et al., 2006; Kirwan and Murray, 2007; Marani et al., 2007; Mariotti and Fagherazzi, 2010; Temmerman et al., 2012). In large lagoons or extensive tidal basins, the low elevation of the tidal flats is mainly maintained by wave erosion, as systematically elaborated in Hu et al. (2015b, 2018). The existence of these two alternative stable states has been empirically observed on the large scale of whole tidal basins where large areas (∼1–10 km2) of marshes and tidal flats may coexist next to each other (Marani et al., 2007; Carniello et al., 2009; Wang and Temmerman, 2013). However, the existence of alternative stable states has not yet been empirically explored to explain marsh loss and recovery by formation and revegetation of bare patches (∼10–100 m2), which is addressed in this paper.
Bare patches are defined here as nonvegetated areas in the interior of otherwise vegetated marshes. Here we consider two types of bare patches: (i) connected bare patches that have a connection to the tidal channel network and (ii) isolated bare patches that are separated from the channels by surrounding marsh vegetation. Literature suggests that unconnected bare patches start as areas with vegetation die-off, by increased flooding stress and inadequate drainage; high salinity stress (DeLaune et al., 1994; Wilson et al., 2009, 2014); coverage by drifted plant material (Harshberger, 1916; Miller and Egler, 1950; Redfield, 1972); physical disturbance by ice; or herbivory by crabs, nutria, muskrats, geese, or snails (Harshberger, 1916; Stevenson et al., 1985; DeLaune et al., 1994; Silliman, 2005; Argow and FitzGerald, 2006). Subsequent elevation loss due to the collapse of the root structure or decomposition and disintegration of soil organic matter can deepen the bare patches (DeLaune et al., 1994; Wilson et al., 2014). Connected bare patches form by creek-bank erosion at the creek heads (Kearney et al., 1988) as well as subsequent connection of channel heads to bare patches (Redfield, 1972) or by expansion of unconnected bare patches that ultimately reach a channel and become hydraulically connected to the channel network (Wilson et al., 2014; Mariotti, 2016).
Nevertheless, under which topographic conditions connected and unconnected bare patches occur, especially under which conditions they recover through re-establishment of vegetation, is not fully understood. For example, we may hypothesize that unconnected bare patches are buffered from tidal currents and waves by the surrounding marsh vegetation and are therefore less prone to erosion and more suitable for vegetation recovery. On the other hand, they might also receive less sediment input since sediment is efficiently trapped by the surrounding vegetation buffer (Mudd et al., 2010; Moskalski and Sommerfield, 2012). The opposite applies for connected bare patches: they receive direct sediment input through the channels but experience higher flow velocities that may cause sediment resuspension and erosion. Some studies show that marsh plants might recolonize bare patches when they become connected, drain, and if vertical accretion elevates the bare patches sufficiently for plant establishment (Redfield, 1972; Wilson et al., 2009, 2014). However, higher flow velocities and therefore a decrease in accretion by reduced mineral sediment deposition or erosion may inhibit the recovery of vegetation in connected bare patches (DeLaune et al., 1994; Mariotti, 2016).
Hence, despite the fact that bare patches are often recognized as symptoms of marsh loss (Kearney et al., 1988; DeLaune et al., 1994; Fagherazzi et al., 2013; Mariotti and Fagherazzi, 2013; Wilson et al., 2014; Mariotti, 2016), there are relatively few studies on the dynamics of bare patches. For example, the modeling study by Mariotti (2016) simulates that pond expansion is favored under conditions with low tidal range, low sediment supply, and high relative sea level rise. Apart from this study, there is poor empirical evidence on the conditions that determine the presence and/or recovery of bare patches, especially across marsh sites that differ in characteristics such as tidal range, sediment supply, and plant species. In this paper, we first study the topographic conditions determining the presence and dynamics of bare patches. Next, we study the topographic conditions determining the marsh vegetation recovery (i.e., the re-establishment of vegetation) in bare patches. To identify the topographic conditions determining the presence of bare patches, we compared the surface elevation, bare patch size, and distance from channels for connected and unconnected bare patches in three different sites, located in the Scheldt estuary (a river-dominated estuary in the Netherlands, 4.8 m tidal range), Venice lagoon (a back-barrier lagoon in Italy, 1.0 m tidal range), and Blackwater marshes (a submerging tidal marsh in Maryland, USA, <0.5 m tidal range). To identify the conditions determining the revegetation of bare patches, we carried out a time series analysis in the Scheldt estuary, which is the only site where revegetation was observed, and searched for relations between the rate of revegetation of bare patches and topographic conditions including surface elevation, distance from channels and the width of connecting channels. Our hypotheses are the following: (1) Bare patches across all three study sites are found at similar elevations relative to the tidal frame, distance from tidal channels, and degree of connectivity to tidal channels (connectivity is defined here as the width of connecting channels); (2) low elevation relative to the tidal frame and wide channel connection lead to larger bare patches that are more difficult to revegetate.
2.1 Saeftinghe marsh, Scheldt estuary, the Netherlands
The Scheldt estuary is a river-dominated estuary located in the southwest of the Netherlands and the northwest of Belgium (Fig. 1). The Saeftinghe marsh (51.33∘ N, 4.17∘ E) is a 3000 ha brackish tidal marsh. It is subject to a semidiurnal tidal regime with a local mean tidal range of 4.88 m, a salinity of 5–18 PSU (practical salinity unit), and a mean suspended sediment concentration (SSC) of 30–60 mg L−1 (Temmerman et al., 2003a; van Damme et al., 2005). In the last 80 years, a long-term rise of mean high water level (MHWL) was observed in the Saeftinghe marsh at a rate of 5.7 mm yr−1, while the vegetated marsh regions expanded in area and increased in elevation steadily and continuously (Wang and Temmerman, 2013). Dominant plant species include Sporobolus anglicus (in earlier literature referred to as Spartina anglica), Salicornia europaea, Scirpus maritimus, Elymus athericus, and Phragmites australis. Marsh vegetation is observed between −2 m and +1 m relative to MHWL (Wang and Temmerman, 2013). Parts of the Saeftinghe marsh have been converted to bare patches. This is partly attributed to geese grubbing for belowground tubers (Elschot et al., 2017). In addition, bare patches are formed at places with poor drainage after high over-marsh tides (i.e., high tides that submerge the complete marsh surface), especially near the head of the smallest tidal channels. This is the case in the selected study site, covering an area of 35 ha (Fig. 1).
2.2 San Felice marsh, Venice lagoon, Italy
The Venice lagoon is a back-barrier tidal lagoon situated in the northeast of Italy, characterized by a micro-tidal semidiurnal regime with a mean tidal range of about 1.0 m (Day et al., 1999) and a maximum tidal range of 1.5 m (Rinaldo et al., 1999a, b; Marani et al., 2007). The long-term rate of relative sea level rise varies around 3–4 mm yr−1 (Carbognin et al., 2004). The marsh area in the Venice lagoon has decreased by about 75 % since 1901, which is caused by both drowning and lateral erosion of marshes (Tommasini et al., 2019). The San Felice salt marsh (45.48∘ N, 12.46∘ E) is one of the best preserved marshes in the Venice lagoon, being capable of keeping pace with current relative sea level rise (e.g., Roner et al., 2016; Marani et al., 2003). The average salinity varies between 24 and 33 PSU (Gieskes et al., 2013; Zirino et al., 2014), and the average SSC is between 10 and 20 mg L−1 (Zaggia and Ferla, 2005; Defendi et al., 2010; Venier et al., 2014). The salt marsh is occupied by halophytic species, such as Salicornia veneta, Sporobolus maritimus (in earlier literature referred to as Spartina maritima), Limonium narbonense, Sarcocornia fruticosa, Puccinellia palustris, Inula crithmoides, and Juncus maritimus (Silvestri et al., 2005; Marani et al., 2006). The elevation of the salt marsh ranges from 0 to 0.7 m relative to mean sea level. Our study site has an area of 72.3 ha (Fig. 2).
2.3 Blackwater marshes, Chesapeake Bay, USA
The Blackwater marshes (38.40∘ N, 76.08∘ W) are located along the Chesapeake Bay (Maryland, USA). They cover an area of about 6000 ha with an average SSC of about 50 mg L−1 and an average salinity of 10 PSU (Stevenson et al., 1985; Ganju et al., 2013; Kirwan and Guntenspergen, 2015). Long-term local sea level rise is currently 3.7 mm yr−1 (NOAA station 8571892, http://tide- sandcurrents.noaa.gov/sltrends, last access: 19 December 2016). About half of the interior marshes have disappeared since 1938, mainly by the development and enlargement of bare patches, which are occurring as interior marsh pools (Stevenson et al., 1985; Kearney et al., 1988; Kirwan and Guntenspergen, 2012; Schepers et al., 2017). The pool expansion has been attributed to submergence by sea level rise, vegetation disturbance by invasive herbivores, and subsequent open-water expansion (Stevenson et al., 1985; Kendrot, 2011). Changes in water level are mainly driven by meteorological events (wind and air pressure), while the astronomical tidal amplitude is about 0.25 m at our study site. Brackish vegetation dominates, with species such as Scirpus americanus, Sporobolus alterniflorus (synonymously known as Spartina alterniflora), Sporobolus pumilis (synonymously known as Spartina patens), Distichlis spicata, Spartina cynosuroides (synonymously known as Spartina cynosuroides), and Phragmites australis (Pendleton and Stevenson, 1983; Kirwan and Guntenspergen, 2012). Our study area covers an area of about 699.8 ha (Fig. 3).
3.1 General procedure
For all three study sites, aerial photographs were digitized, georeferenced, and manually classified into vegetated marshes, unconnected bare patches, connected bare patches, and tidal channels (Figs. 1–3). Bare patches that were smaller than 1 m2 were not considered in this study. Given the resolution of the images (see below), bare patches were classified as to be connected to the channel network when the connecting channel was at least 0.5 m wide. Hence our classification of unconnected bare patches may also include patches with a small connecting channel (less than 0.5 m wide). The edge between a connected bare patch and the connecting channel was visually defined as where the channel planform shape (i.e., linearly shaped) in upstream (landward) direction widens into a bare patch (i.e., nonlinear, more irregular shape), as shown in Figs. 1–3. Lidar data were used to analyze the elevation differences between vegetated marshes, unconnected bare patches, connected bare patches, and tidal channels. When bare patches were inundated during the lidar survey, the soil surface elevation within the bare patches was measured with field surveys (methods are explained below for the different study sites). Generally, lidar data have larger and more homogeneous spatial coverage and higher spatial resolution. Field surveys only include selected locations, but field surveys of soil surface elevation had greater vertical accuracy than lidar surveys (see below), especially for vegetated areas where lidar partially reflects on the vegetation canopy and open water where lidar reflects on the water surface. All the spatial analyses were done using ArcGIS.
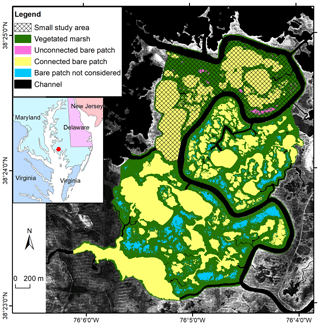
Figure 3Study area in the Blackwater marshes. Spatial distribution of vegetated marshes, unconnected bare patches, and connected bare patches in 2010 with lidar images as background. Data presented in this paper are for all bare patches in the small study area – both unconnected ones (in pink) and connected ones (in yellow). In order to obtain a higher number of observations of connected bare patches, we also included connected bare patches in the larger study area (in yellow) but excluded unconnected bare patches (in blue).
3.2 Saeftinghe
For the Saeftinghe study site, a time series of false-color aerial images (Fig. S1 in the Supplement) was used, from 1990, 1998, 2004, and 2008. The four images were selected considering the data availability and to detect dynamic changes from vegetated marsh portions into bare patches and vice versa. All the photos were processed in a similar way, by scanning, georeferencing, and mosaicking them into digital pictures with a minimum resolution of 0.5 m. All the aerial images were provided by Rijkswaterstaat (the Dutch governmental institute for water management) (Huijs, 1995; van der Pluijm and de Jong, 1998; Reitsma, 2006; Bakker and Bijkerk, 2009). From all the available aerial photographs, we extracted two sample areas (Fig. 1) free from drifted plant debris, which were analyzed together. The digitized aerial images in the sample areas were classified into vegetation, water, and bare soil based on supervised maximum likelihood classification and then further classified visually into vegetated marshes, channels, connected bare patches, and unconnected bare patches. For elevation data in Saeftinghe, we used a digital terrain model (DTM) with a resolution of 2×2 m, which was obtained from a lidar survey performed in 2004 during low tide with a maximum vertical error of 0.2 m (Alkemade, 2004). The measurement point density of the lidar survey varied from one point per 16 m2 to several points per 1 m2. The DTM data were also provided by Rijkswaterstaat. We used only one lidar dataset to derive the elevations of bare patches and marshes over the period 1990–2008, because previous research in the area showed that during that period elevation changes were limited with maximum rates of 1 cm yr−1 (Wang and Temmerman, 2013). This implies that over the considered timescale (1990–2008), maximum elevation changes (∼18 cm in 18 years) are of the same order of magnitude as the vertical error of the lidar data (∼20 cm). Therefore, we decided to use one lidar-based DTM for 2004, which is considered to be representative to characterize the approximate time-averaged elevation of marshes and bare patches over the period 1990–2008. No field survey data were used for Saeftinghe since all bare patches drain completely during neap tides so that soil surface elevations were recorded by lidar.
3.3 San Felice
For the San Felice study site, our analysis was based on a vegetation map classified from a hyperspectral image with a resolution of 1.3 m, which was acquired in 2002 by the airborne CASI sensor (15 bands in the visible and near-infrared portion of the spectrum) (Belluco et al., 2006). It was visually reclassified into channels, connected bare patches, unconnected bare patches, and vegetated marshes (Fig. 2). For the latter, we consulted a black and white aerial photograph acquired in 2000 with a resolution of 16 cm and a 1 m resolution pan-sharpened multispectral Ikonos satellite image acquired in 2006 (Fig. S2). For elevation data in San Felice, we used both a DTM obtained from a lidar survey and field measurements. The lidar survey was performed during low tide in 2002 with a mean measurement point density of about 48 points per 1 m2 and a vertical accuracy better than 0.15 m (Wang et al., 2009). From these data, we constructed a gridded DTM with a spatial resolution of 1×1 m. Field elevation measurements from the Venice Water Authority in 2000 were also used, because some bare patches were inundated during the lidar survey. Data were collected with stereo aerial photography for marshes, stadia rods with GPS for areas close to marshes and mudflats, and single-beam echo sounder for shallow waters (Sarretta et al., 2010). In total, 340 elevation measurements were located in vegetated marshes, and 95 measurements in bare patches. The boundary of the study area was delineated by channels and creeks as shown in Fig. 2, considering the availability of data. Since almost no vegetation recovery in bare patches was observable on aerial images from the San Felice marsh, we did not do a time series analysis on vegetation recovery.
3.4 Blackwater
In the Blackwater study site, we selected a study area away from the influence of roads and uplands (Fig. 3). The small study area (marked with shading in Fig. 3) was chosen for the field survey. A larger study area (the entire colored region in Fig. 3) was later considered in order to increase the number of bare patches connected to channels wider than 1 m. Bare patches that are connected with narrow channels (<1 m) and that are located outside of the small study area (blue polygons in Fig. 3) were not considered in the analysis. We used false-color aerial photographs with a spatial resolution of 0.3 m obtained in 2010 (Fig. S3) and provided as digitized and georeferenced mosaics by the United States Department of Agriculture (USDA). We classified the photos into vegetated marshes, connected bare patches, unconnected bare patches, and channels, using the same method for Saeftinghe. We also used data acquired from a lidar survey and a field survey. The lidar data were obtained in 2003 with an average area sampling density of about 0.8 points per 1 m2 and a mean vertical accuracy of 0.14 m. The DTM was provided with a resolution of 2×2 m by the US Geological Survey and Maryland Department of Natural Resources. As most bare patches were covered by water during the lidar survey, a field survey was carried out in 2012 in the small study area using RTK-GPS (real-time kinematic GPS) with ±1.5 cm accuracy. In total, 36 elevation measurements were collected in five unconnected bare patches, 31 measurements in five connected bare patches, and 93 measurements in the vegetated marshes. An overview of the number of data points (lidar and GPS measurements) that fall within marshes and bare patches are given for the different study sites in Table 1. We did not do a time series analysis on vegetation recovery, because other studies have demonstrated that recovery is absent (Schepers et al., 2017).
4.1 Topographic conditions determining the presence of bare patches
In order to identify the topographic conditions determining the presence of bare patches or marsh vegetation, we analyzed the frequency distributions of surface elevation and distance from channels for connected and unconnected bare patches, and we compared them with the vegetated marsh portions for the three study sites. The surface elevation was analyzed using lidar data and field data. The distance from channels was calculated as the Euclidean distance from the edge of channel polygons. Bare patches smaller than 1 m2 were excluded from the analysis. The nonparametric Mann–Whitney U test (R Core Team, 2016) was conducted to test whether or not there were significant differences between elevations of vegetated marsh portions and connected and unconnected bare patches.
Elevation classes of 10 cm were used, since smaller elevation classes were not deemed to be reasonable considering the vertical accuracy of the lidar data. Surface elevation relative to the tidal frame, which is defined here as the elevation range between the local mean low and high water levels, is an important factor for vegetation, because it determines the frequency, depth, and duration of tidal flooding and is widely considered a crucial ecological condition for marsh plant growth (e.g., Balke et al., 2016). Therefore, in order to allow for comparisons between the three marsh sites with largely different tidal ranges, we rescaled the surface elevation relative to the tidal frame using the following relationship:
where RE is the relative elevation (a dimensionless proportion of the local tidal frame), E is the actual elevation (in meters relative to a fixed datum), and MLWL and MHWL are the mean low water level and mean high water level, respectively (in meters relative to the same datum). Hence RE is 0 for elevations equal to MLWL and is 1 for elevations equal to MHWL.
In addition, the frequency distribution of bare patch sizes was calculated and related to the widths of channels that were connected to bare patches. The channel width was measured on the aerial photographs at the connection with the bare patch for each single patch and classified into categories with 5 m spacing. Unconnected bare patches (channel width < 0.5 m) and bare patches connected with small channels (channel width between 0.5 and 1 m) were classified as two separate categories because of their large number. We combined all bare patches with a connection > 80 m in the highest class, since there were only zero, one, and two patches for this category in the Saeftinghe, San Felice, and Blackwater marsh sites, respectively.
4.2 Topographic conditions determining the revegetation of bare patches in Saeftinghe
We studied revegetation of bare patches in the Saeftinghe marsh during the last 2 decades. We did not include the San Felice and Blackwater marshes in this analysis, because there was almost no revegetation recognizable on the aerial photographs during this period of the last 2 decades. Between each aerial photograph in 1990, 1998, 2004 and 2008, we identified areas that changed from vegetated to bare surfaces, areas that revegetated from bare to vegetation, and areas that remained bare or vegetated. From this data, we determined the rate of revegetation of bare areas. We made a distinction between the following classes:
-
permanent bare patches that never revegetated within the considered time period from 1990 to 2008;
-
rapidly revegetated bare patches, identified as bare in only one image, either 1998 or 2004, and observed as vegetation in the other three images;
-
permanent marsh areas, classified as vegetation throughout the time series.
In order to identify the topographic conditions for rapid or no revegetation of bare areas, the frequency distribution of elevation was calculated for these three classes (permanent bare patches, rapidly revegetated bare patches, and permanent marsh areas), as well as the frequency distributions of the distance from the closest channel. In addition, we also determined the width of the channels connecting to the bare patches. For permanent bare patches, the channel width is calculated as the mean value for 1990, 1998, 2004 and 2008. For rapidly revegetated bare patches, the channel width is the value when the bare patches occurred, either in 1998 or in 2004. In order to identify the relationship between the rate of revegetation and the width of connecting channels, the frequency distribution of channel widths was compared between permanent bare patches and rapidly revegetated bare patches.
5.1 Topographic conditions determining the presence of bare patches
In order to identify the topographic conditions determining the presence of bare patches, we tested relationships between their presence and three topographic variables, which are (1) elevation of the bare soil surface, (2) distance of the bare patches from channels, and (3) channel width for bare patches connected to channels (channel width < 0.5 m for unconnected bare patches). We first tested whether these three topographic variables are independent from each other. The correlations were low (Pearson's r<0.5) and not significant (p>0.05) between all variables and for all field sites. Only for the Blackwater marsh was the correlation between the elevation and the channel width high (Pearson's ), but this correlation is based on a very low number of connected (n=5) and unconnected (n=5) bare patches.
5.1.1 Elevation
In Saeftinghe, the connected bare patches, unconnected bare patches, and vegetated marshes fall within the elevation ranges of 2.3–3.5 m a.m.s.l. (above mean sea level), which is close to the local MHWL (relative elevation RE=0.91–1.16) (Fig. 4a). The differences in elevation between the vegetated marshes and connected and unconnected bare patches were statistically significant between all comparisons of two of the three variables (p<0.001 based on the Mann–Whitney test). The peaks of the elevation distribution (i.e., the mode of the elevation distribution) for the vegetated marshes and unconnected bare patches are 0.1 m higher than for the connected bare patches (or the difference between relative RE, ΔRE=0.02). The mean elevation of the vegetated marshes is highest (2.97 m a.m.s.l., RE=1.05), whereas this is 0.14 m lower for the unconnected bare patches (ΔRE=0.03) and 0.23 m lower for the connected bare patches (ΔRE=0.05).
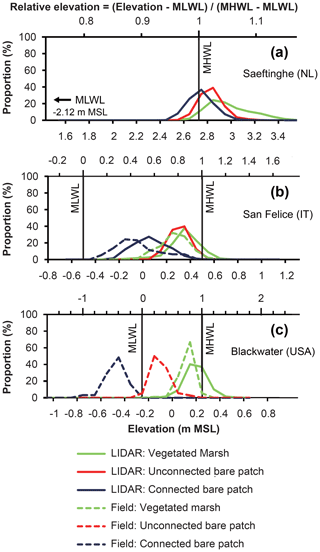
Figure 4Elevation distribution of vegetated marshes, unconnected bare patches, and connected bare patches based on lidar surveys and field surveys for (a) Saeftinghe, (b) San Felice, and (c) Blackwater. In each figure, the bottom x axis shows the absolute elevation (in meters relative to mean sea level) and the top x axis shows the relative elevation (dimensionless, as defined in Eq. 1). The proportion on the y axis is calculated based on lidar or field measurements as the number of pixels or samples in each elevation class (every 0.1 m) relative to the total number of pixels or samples for each category. The total numbers in each category are given in Table 1. The MLWL in Saeftinghe is 2.12 m lower than m.s.l., which is outside of the range of the main x axis in (a). MLWL and MHWL definitions at Blackwater are approximate since water level changes are dominated by meteorological rather than astronomical influences. Field data were added to lidar data for San Felice and Blackwater, because bare patches were partly covered there by water, which obstructs lidar sensing of the soil surface beneath the water surface. In Saeftinghe all bare patches were drained at low tides, and lidar measurements are not obstructed here by water cover.
In San Felice, the connected bare patches, unconnected bare patches, and vegetated marshes are situated in different ranges of elevations between −0.5 and +0.7 m relative to m.s.l. (RE=0–1.2, Fig. 4b). The differences in elevation distributions of these three categories are also statistically significant (p<0.001 based on the Mann–Whitney test). The elevation measured in the field is lower than that from the lidar survey for both the connected bare patches and vegetated marshes. The peaks of the elevation distribution of the vegetated marshes and unconnected bare patches are about 0.15 m lower than MHWL (RE=0.85) based on lidar data, and about 0.3 or 0.5 m higher than connected bare patches (ΔRE=0.3 or 0.5) based on lidar or field data, respectively. The mean lidar elevation of the vegetated marshes is 0.35 m relative to m.s.l. (RE=0.85), which is 0.04 m higher than unconnected bare patches (ΔRE=0.04) and 0.28 m higher than connected bare patches (ΔRE=0.28).
In the Blackwater marshes, connected bare patches, unconnected bare patches, and vegetated marshes occupy significantly different ranges of elevations (p<0.001 based on Mann–Whitney test) between −0.7 and +0.5 m relative to m.s.l. (–1.5, Fig. 4c). The peaks of the elevation distribution of the vegetated marshes are 0.1 m lower than MHWL (RE=0.8), 0.3 m higher than unconnected bare patches (ΔRE=0.6), and 0.6 m higher than connected bare patches (ΔRE=1.2). The mean elevation is the highest for the vegetated marshes (0.13 m relative to m.s.l., RE=0.76), 0.23 m lower for the unconnected bare patches (ΔRE=0.46), and 0.6 m lower for connected bare patches (ΔRE=1.2).
Together these results indicate that connected bare patches, unconnected bare patches, and vegetated marshes tend to occupy different elevation ranges at each site (p<0.001 by Mann–Whitney), with the largest absolute elevation differences in Blackwater, the smallest in Saeftinghe, and intermediate values for San Felice. Connected bare patches always lie within the lowest elevation range, whereas vegetated marshes always dominate the highest elevation range around MHWL. Unconnected bare patches are always found in the intermediate elevation range, which is about 0.1–0.5 m higher than the connected bare patches. The difference in RE (relative to the tidal frame) between the connected and unconnected bare patches is about 0.02 in Saeftinghe, 0.2–0.5 in San Felice, and 0.6–0.8 in Blackwater.
5.1.2 Distance from channels
The frequency distribution of the distance between a bare patch and closest channel shows similar results for the three marsh sites (Fig. 5). Vegetated marshes rather than bare patches occur near channels. With increasing distance from channels, marsh vegetation becomes less frequent and unconnected bare patches become more frequent. Connected bare patches occur most frequently at large distances from the channels. The peak of the distribution is situated at 1.0 m for vegetated marshes in all three sites; at 8 m for unconnected bare patches and over 10 m for connected bare patches in both Saeftinghe and San Felice; and at 82 and 89 m for unconnected and connected bare patches in Blackwater, respectively.
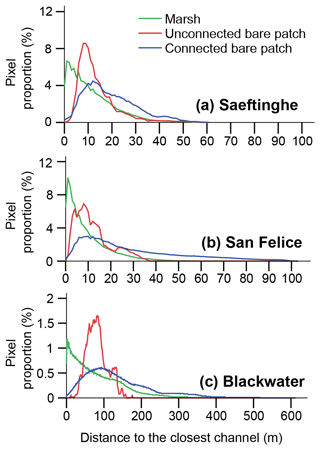
Figure 5Frequency distribution of distances to the closest channel in (a) Saeftinghe, (b) San Felice, and (c) Blackwater. The proportion is calculated as the number of pixels in each distance class (every 1 m) relative to the total number of pixels for each category, i.e., vegetated marshes, unconnected bare patches, or connected bare patches.
5.1.3 Bare patch size in relation to connectivity to channels
Bare patch size generally increases with increasing width of connecting channels, whereas the number of bare patches decreases with increasing channel widths (Fig. 6). The unconnected bare patches in Saeftinghe, San Felice, and the small study area of Blackwater occupy 63 %, 36 %, and 67 % of the total number of bare patches, respectively, but only 2 %, 1 %, and 3 % of the total area of bare patches, respectively. Hence, unconnected bare patches are numerous but small. The number of connected bare patches, in contrast, is in most cases smaller, and they become less abundant with increasing width of the connecting channels.
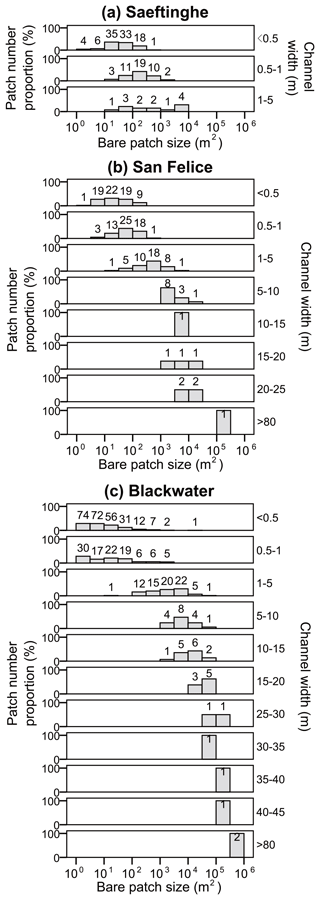
Figure 6Frequency distribution of bare patch sizes in relation to the connected channel width in (a) Saeftinghe, (b) San Felice, and (c) Blackwater. Note the x axis is in logarithmic scale. Bare patches with connecting channel widths < 0.5 m are defined as unconnected bare patches in the text (see Sect. 3.1). The patch number proportion (%) is calculated as the number of bare patches in each class of bare patch size relative to the total number of bare patches for each category of channel width. The number of bare patches in each size class is labeled at the top of the bars.
5.2 Topographic conditions determining the revegetation of bare patches in Saeftinghe
The multitemporal analysis for Saeftinghe shows that bare patches have been dynamically expanding or shrinking between the four images of 1990, 1998, 2004, and 2008 (Fig. S4). We focused on bare areas with two extreme rates of revegetation, which are permanent bare areas (which never revegetated throughout the time series) and rapidly revegetated bare areas (only present in 1998 or 2004 and revegetated by the next time step). The spatial distribution of these bare categories (Fig. S4) suggests that the inner portion of big connected bare patches tends to be stable and never revegetated within the studied period, while rapidly recovering bare areas are mainly present at the edge of small bare patches.
5.2.1 Elevation
The elevation distribution showed that permanently bare areas (i.e., remaining bare over the studied 18-year period) occupy the lowest range of elevations, whereas permanent marsh areas have the highest range of elevations (Fig. 7a). At intermediate elevations, bare patches become rapidly revegetated (i.e., within 4 to 6 years after their first appearance) (Fig. 7a).
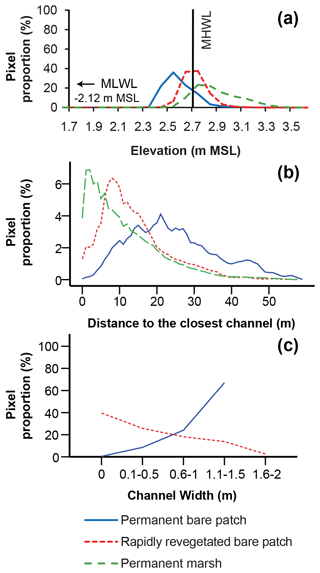
Figure 7Frequency distribution of (a) elevation, (b) distances to the closest channel, and (c) the connected channel width for permanent bare patches, rapidly revegetated bare patches, and permanent marsh areas in Saeftinghe. The elevation is relative to mean sea level and binned into 0.1 m intervals. The proportion in panel (a) is calculated as the number of pixels in each elevation class (every 0.1 m) relative to the total number of pixels for each category. The proportion in panel (b) is calculated as the number of pixels in each distance class (every 1.0 m) relative to the total number of pixels for each category. The proportion (%) in panel (c) is calculated as the number of pixels in each class of channel width relative to the total number of pixels that are permanently bare patches (blue line) or rapidly revegetated bare patches (dashed red line).
5.2.2 Distance from channels
The frequency distribution of the different bare categories with distance from the channels (Fig. 7b) shows that stable marshes are closest to channels with a peak around 1–2 m from channels. Bare areas that revegetated quickly have an intermediate distance around 8 m from channels, whereas permanent bare areas are located farthest from the channels with a peak at 21 m.
5.2.3 Connectivity to channels
Permanent bare areas are always connected to channels and tend to be associated with wide channels, while unconnected bare patches always rapidly revegetated (i.e., within 4 to 6 years after their first appearance) (Fig. 7c). The percentage of bare areas that become revegetated increases with decreasing channel width (Fig. 7c).
Bare patches within otherwise vegetated coastal marshes are often recognized as symptoms of marsh loss in many places around the world (Kearney et al., 1988; Fagherazzi, 2013; Mariotti and Fagherazzi, 2013; Ortiz et al., 2017; Schepers et al., 2017), but comparative studies among different marsh systems to better understand the conditions that determine their presence and potential vegetation recovery are relatively scarce (e.g., Mariotti, 2016). For three marsh sites with different tidal ranges, sediment input, and plant species, we showed that (1) bare patches connected to channels occur most frequently at the lowest surface elevations and farthest distances from creeks; unconnected bare patches most frequently occupy intermediate elevations and distances from creeks, and they are smaller in size and larger in number; and vegetated marshes dominate at the highest surface elevations and closest to creeks. (2) The elevations of connected and unconnected bare patches tend to be lower relative to the tidal frame in sites with a smaller tidal range, although our analysis only included three sites. (3) Recovery of vegetation in bare patches at the timescale of the last 2 decades was only observed in the site with high tidal range and high sediment input (Scheldt estuary). No vegetation recovery was observed in the two sites with smaller tidal range and sediment input (Venice lagoon, Blackwater marshes), which is in line with previous studies in these two areas, showing progressive marsh die-off over longer (century) timescales (e.g., Schepers et al., 2017; Carniello et al., 2009). Here vegetation recovery is hampered by low surface elevations relative to the local tidal frame, by farther distance from channels and by wider channels connecting the bare patches to the channel network. Below we will further substantiate these findings and discuss interpretations and potential hypotheses that may explain mechanisms of formation and recovery of bare patches.
6.1 Topographic conditions determining the presence of bare patches
Our results suggest that bare patches exist under qualitatively similar topographic conditions across three different marsh systems. We found that marshes have a higher elevation than bare patches, in accordance with previous studies (DeLaune et al., 1994; Erwin et al., 2006; Wilson et al., 2014). In addition to existing insights, we found that unconnected bare patches are most frequently found at higher elevations and shorter distances from channels as compared to connected bare patches (Figs. 4 and 5). Additionally, we found a positive relationship between patch size and the width of the connecting channel (Fig. 6). These different observations may be interpreted as follows. First, concerning the positive relationship between bare patch size and connecting channel width, we are not certain about the direction of causal relationship (either larger bare patches causing wider connecting channels or vice versa), but we may formulate certain hypotheses. This relationship may be due to the difference in tidal prism (i.e., the total water volume that floods into and drains out of the bare patches during a tidal cycle). A larger bare patch implies a larger tidal prism, which means that higher volumes of water are transported into and out of the bare patches. Assuming that most of the water is transported through the connecting channel, a larger tidal prism would be associated with larger channel-forming discharges and therefore wider channels (e.g., Rinaldo et al., 1999b; Kirwan et al., 2008; D'Alpaos et al., 2010; Vandenbruwaene et al., 2013).
Secondly, our finding that unconnected bare patches occur most frequently at higher elevations than connected bare patches may be interpreted by a number of potential hypotheses. We expect that connected bare patches experience higher incoming and outgoing flood and ebb flow velocities as they are directly connected to the channels, while unconnected bare patches are surrounded by marsh vegetation, which is expected to obstruct and reduce flood and ebb flow velocities. Furthermore, the time lag between incoming and outgoing tides can result in ebb dominance in marshes (e.g., Friedrichs and Perry, 2001) and therefore may contribute to net sediment export from bare patches that are connected to the channel network. As such, stronger tidal currents, ebb dominance, and net sediment export may result in lower surface elevation of connected bare patches as compared to unconnected bare patches, where the surrounding vegetation may reduce flow velocities and facilitate the deposition of suspended sediments supplied during over-marsh tides. Such effects of tidal currents may be most pronounced in the study site with largest tidal range (Saeftinghe), while additional effects of wind waves on sediment transport have been reported to be important in the sites with intermediate and small tidal range (San Felice, Blackwater) (e.g., Stevenson et al., 1985; Fagherazzi et al., 2006). We found that connected bare patches are larger (Fig. 6); hence, we may expect more potential for erosion of surface sediments induced by waves (because of larger wind fetch length). Wave erosion in interior marsh ponds has been found to be related to the size and wind fetch length of marsh ponds (Mariotti and Fagherazzi, 2013; Mariotti, 2016; Ortiz et al., 2017). Hence larger bare patches are likely to experience more wave-induced erosion and are found in this study to be connected through wider connecting channels, which may facilitate the tidal export of the eroded sediments from connected bare patches and therefore may explain the lower surface elevation of connected bare patches. In contrast, we hypothesize that unconnected bare patches, which are typically smaller (Fig. 6), may be expected to experience less wave erosion (smaller fetch length) and much weaker flow velocities (as flow is obstructed by surrounding vegetation). With respect to the latter effect, we notice that our classification of unconnected patches may also include patches with connecting channels smaller than 0.5 m but impossible to detect on the aerial images. Nevertheless, also in the case of such small connecting channels < 0.5 m wide, one can expect that drainage of the bare patches after over-marsh tides is much slower, with lower ebb flow velocities, as compared to bare patches with wide connecting channels (up to several tens of meters wide; see Fig. 6), facilitating faster drainage, higher ebb flow velocities, and potentially leading to larger tidal export of eroded sediments. Unconnected bare patches were also found to occur most frequently at shorter distances from channels as compared to connected bare patches, and this may facilitate higher sediment supply to unconnected bare patches closer to channels, as suspended sediment concentrations typically decrease with increasing distance from channels (Leonard, 1997; Christiansen et al., 2000; Temmerman et al., 2003b). Therefore, higher sediment supply and lower magnitude of waves and tidal currents in smaller, unconnected bare patches at shorter distance from channels may facilitate the settlement of suspended sediments and reduce erosion, and as such this may explain our finding of higher surface elevations of unconnected bare patches as compared to connected bare patches. This finding is also in accordance with the model of Mariotti (2016), proposing that, in what is called the “pond collapse regime”, the depth of connected marsh ponds would be larger than the depth of unconnected ponds.
Thirdly, our results indicate that connected bare patches are predominantly located farther away from channels than unconnected bare patches (Fig. 5). One potential explanation is that connected bare patches are generally larger than unconnected bare patches (Fig. 6), so a larger fraction of the connected bare patches is located at a farther distance from channels. The presence of bare patches in relation to distance from channels has been previously studied on large regional scales (102–104 m), considering only large estuarine channels (Turner and Rao, 1990; Kearney and Rogers, 2010). On a smaller scale (10–102 m), Redfield (1972) qualitatively reported that big bare patches are located relatively far from channels. Adamowicz and Roman (2005) observed that bare patches were located at around 11 m from the nearest channel in both ditched and unditched marshes in New England. Such a value is similar to that found for Saeftinghe and San Felice but smaller than the value obtained for Blackwater. The elevation difference between connected and unconnected bare patches probably relates to their difference in distance from channels. Marshes typically have a micro-topography of higher levees along channels and lower depressions farther away from channels as a consequence of progressive suspended sediment deposition during tidal flooding of marshes from channels (e.g., Reed, 1988; Covi and Kneib, 1995; Leonard, 1997; Esselink et al., 1998; Reed et al., 1999; Allen, 2000; Temmerman et al., 2004; D'Alpaos et al., 2007; Bartholdy, 2012). In accordance with this micro-topography, the lower-elevation connected bare patches are located farther away from channels than the higher-elevation unconnected bare patches. This micro-topography of levees close to channels and depressions further away from channels is often associated with an increasing inundation duration after high tides and decreasing soil drainage/aeration during low tides, with increasing distance from channels (e.g., Ursino et al., 2004). Also, a modeling study suggested that marsh vegetation expansion can lead to increased inundation time, and as such can feed back on increased stress and chance for vegetation dieback (Brückner et al., 2019). These may all be mechanisms that contribute to increased chance for occurrence of bare patches within marshes at farther distances from channels. In addition, the frequency distribution of distance from the closest channels is observed to be exponential for the vegetated marsh surfaces in all three marsh sites, which is analogous to the results by Marani et al. (2003) and holds only for the vegetated marsh surfaces.
Finally, our results demonstrate that the size of bare patches is negatively related to the number of bare patches (Fig. 6). Such a finding has also been observed in other marsh systems (Turner and Rao, 1990; Schepers et al., 2017). This may be indicative for initial formation of many small bare patches that grow and merge together through time, hence leading to a decreasing number of larger patches. This process of merging of initially small bare patches into larger patches has been documented for the Blackwater study site from an analysis of time series of aerial photos over the period 1938–2010 (Schepers et al., 2017).
In conclusion, we observed qualitatively similar topographic conditions for the presence of bare patches across the three study sites, although elevations of connected and unconnected bare patches tend to be lower relative to the tidal frame in sites with a smaller tidal range. The latter point agrees with earlier findings that micro-tidal marshes have in general a lower surface elevation than macro-tidal marshes (Kirwan et al., 2010; D'Alpaos et al., 2011). Our finding suggests that feedback mechanisms between vegetation and topography are important in regulating the position of the bare patches, and they are perhaps generalizable across systems. However, we emphasize that our analysis is based on only three study sites, and more research is needed to assess the degree to which this finding is universal.
6.2 Topographic conditions determining the revegetation of bare patches
The comparison between bare patches with two extreme revegetation rates (i.e., permanent bare patches over the studied 18-year period and rapidly revegetated bare patches within 4–6 years) for Saeftinghe suggests that fast revegetation preferentially occurs by expansion of the vegetated edge into small (higher elevation) unconnected bare patches, whereas the central areas of big (lower elevation) connected bare patches tend to remain unvegetated over the considered time period of 18 years. These results are consistent with previous studies. For example, only small bare patches were invaded by vegetation in ditched marshes in Louisiana, USA, although large bare patches were permanent over a study period of 22 years (Turner and Rao, 1990). In several New England marshes, re-establishment of vegetation started within 1–2 years after unconnected bare patches merged with the channel network and became drained (Wilson et al., 2009, 2014). Additionally, some studies found that unconnected bare patches expand and merge quickly, while connected bare patches are relatively stable (Kearney et al., 1988). These disparate observations in different marsh sites may be due to different environmental conditions, such as differences in relative sea level rise, tidal range, and sediment availability (Mariotti, 2016). In a modeling study, Mariotti (2016) demonstrated that vegetation recovery in marsh ponds is favored under conditions of slow relative sea level rise, large tidal range, and large inorganic sediment supply.
6.3 Vulnerability for bare patch formation and resilience for bare patch recovery
Previous modeling has suggested that pond formation increases and pond recovery decreases in marsh sites that are subject to a lower suspended sediment availability, smaller tidal range, and lower rate of relative sea level rise (RSLR) (Mariotti, 2016). First of all, we want to emphasize that we only investigated three sites, which is not enough to fully assess the impact of site differences, such as in tidal range, sediment supply, and rate of RSLR, on the occurrence and revegetation of bare patches. Yet we notice that revegetation only occurred at the Saeftinghe site with largest tidal range and sediment supply, while it was not observed at the two other sites (San Felice and Blackwater) with smaller tidal range and sediment supply. As explained in the description of the three study sites (see Sect. 2), the average tidal range and suspended sediment concentrations vary from highest in the Saeftinghe marsh (4.9 m and 30–60 mg L−1, respectively), via intermediate in San Felice marsh (1 m and 10–20 mg L−1, respectively), to lowest in the Blackwater marshes (0.5 m and 50 mg L−1, respectively). Long-term RSLR rates in the San Felice and Blackwater marshes are within the same range of 3–4 mm yr−1, while mean high water level rise in Saeftinghe is 5.7 mm yr−1. In Saeftinghe, marsh elevations are mostly above MHWL, while they are mostly below MHWL in San Felice and Blackwater (Fig. 4). It is probably a combination of all these factors that may explain why the proportion of bare surface area is larger in San Felice and Blackwater (34.33 % and 42.58 %, respectively) than in Saeftinghe (15.72 %).
Marsh resilience inferred by revegetation of bare patches was only observed in Saeftinghe where the mean tidal range is 4.9 m, and bare patches have high elevations relative to the tidal frame (average RE=1.002 for connected bare patches and average RE=1.02 for unconnected bare patches; Fig. 4). Revegetation of bare patches has been observed in other systems with high tidal ranges (Millette et al., 2010; Wilson et al., 2014), which facilitates well-drained conditions during low tide and enables vegetation regrowth. In contrast, in Blackwater where the mean tidal range is about 0.5 m, bare patches have a much lower elevation relative to the tidal frame, even below the MLWL (average for connected bare patches and average RE=0.284 for unconnected bare patches; Fig. 4), which means that there is no drainage at low tide, so marsh vegetation cannot recover. Bare patches also tend to be permanent in other systems under low tidal ranges, such as in Louisiana and mid-Atlantic US salt marshes (Wilson et al., 2014; Ortiz et al., 2017). Clearly, the same elevation loss in a marsh with small tidal range will result in a higher increase in tidal inundation frequency and duration, and this will consequently result in more stress on vegetation growth, as compared to a marsh with a large tidal range. Hence, if marsh vegetation and elevation loss occur, it would be easier to recover marsh vegetation in a higher-tidal-range environment, such as that of Saeftinghe, as compared to situations with a lower tidal range, such as the Blackwater and San Felice marshes. This interpretation is in agreement with previous studies. Micro-tidal marshes were reported to be particularly vulnerable to bare patch formation and expansion (Kearney et al., 1988; Mariotti and Fagherazzi, 2013). Marshes with larger tidal ranges also have bare patches, but they are generally more dynamically forming and recovering, while the whole marsh system is relatively stable (Redfield, 1972; Wilson et al., 2009). The model of Kirwan and Guntenspergen (2010) suggested that extensive bare patches occur, expand quickly, and become permanent under small tidal ranges but not under large tidal ranges, because the elevation range suitable for vegetation growth is smaller in low-tidal-range environments. In general, marsh stability is positively related to tidal range (Kirwan et al., 2010; D'Alpaos, 2011), and numerical modeling indicates that high sediment concentrations are necessary for recovery of bare patches (Mariotti, 2016). However, Mariotti (2016) only considers recovery after connection to the tidal channel network not the recovery of isolated bare patches. In our study, we observed that bare patches unconnected to the tidal channel network all recovered at the Saeftinghe site. Complete drainage of the Saeftinghe bare patches during ebb tides might explain this apparent discrepancy. We suggest that the close distance from channels (see Fig. 5) (e.g., Ursino et al., 2004) and coarser sediment associated with channel levees (Allen, 2000) enable the unconnected bare patches to drain completely in Saeftinghe through subsurface drainage, and this allows for vegetation recovery.
Finally, our results may be useful to decision makers on salt marsh management, as the formation of bare patches may be indicative for marsh degradation towards an unvegetated state that may be difficult to recover. Our study indicates that early signatures for marsh degradation must be particularly monitored in marsh portions, farthest away from main channels, and with lowest surface elevations. Monitoring of early signatures is especially advised in systems with very low tidal range and suspended sediment availability.
In this paper, we studied the topographical conditions for presence and revegetation of bare patches within three coastal marsh sites that are largely different in tidal range, sediment supply, and plant species. The analyses of aerial photographs, lidar data and field topographic measurements showed that the topographic conditions (i.e., elevations, distances from channels, and connectivity to channels) for presence of bare patches were qualitatively consistent among the three marsh sites. We found that bare patches connected to channels occur most frequently at the lowest surface elevations and farthest away from creeks; unconnected bare patches most frequently occupy intermediate elevations and distances from creeks, and they are smaller in size and larger in number; and vegetated marshes dominate at the highest surface elevations and closest to creeks. Further, we showed that the elevations of connected and unconnected bare patches tend to be lower relative to the tidal frame with increasing tidal range, although our analysis only included three sites. Revegetation of bare patches was only observed in one site, which was the site with the highest tidal range and the largest sediment supply. For that site, we found that the chance of bare patch revegetation decreases with increasing width of channels that connect bare patches to the tidal channel network. The latter point is associated with lower bare patch elevation, farther distance from channels, and bigger bare patch size. Finally, in the context of sea level rise, our results suggest that the marsh site with the highest tidal range and highest sediment input is less vulnerable to bare patch formation and more resilient in terms of revegetation of bare patches than the two other marsh sites with lower tidal range and lower sediment supply. However, we emphasize that our study only included three sites, and further research comparing many more sites is needed to further advance our understanding of why certain marsh sites are more vulnerable to formation and persistence of bare patches compared to others. Such knowledge will be important to inform decision makers on site-specific priorities for marsh conservation.
The aerial images and DTM data for Saeftinghe can be downloaded from Rijkswaterstaat (https://www.rijkswaterstaat.nl/zakelijk/open-data, last access: 6 February 2021) (Rijkswaterstaat, 2021). The Ikonos data for San Felice can be accessed at Planetek Italia s.r.l. (https://www.planetek.it/prodotti/tutti_i_prodotti/ikonos, last access: 6 February 2021) (planetek italia, 2021). The aerial images for Blackwater can be downloaded from https://earthexplorer.usgs.gov/ (last access: 6 February 2021) (USGS, 2021). The lidar data for Blackwater can be downloaded from https://www.fisheries.noaa.gov/inport/item/49781 (last access: 6 February 2021) (OCM Partners, 2021). The remaining datasets can be accessed upon request from the first author.
The supplement related to this article is available online at: https://doi.org/10.5194/esurf-9-71-2021-supplement.
ST and CW designed the study. CW prepared the article with contributions from all co-authors.
The authors declare that they have no conflict of interest.
We dedicate this paper to the late Jose Busnelli, who contributed to parts of the analyses presented in this paper. We thank Rijkswaterstaat (Dick De Jong) in the Netherlands, the Venice Water Authority in Italy, and the United States Department of Agriculture and the Maryland Department of Natural Resources in the USA for providing vegetation maps, aerial photographs, and elevation data of lidar surveys and field surveys. We would also like to thank Glenn Guntenspergen, Melissa Duvall, Patrick Brennand, and Kyle Derby for the field measurements in the Blackwater marshes.
This research has been supported by the Joint Research Project of NSFC, NOW, and EPSRC (grant nos. 51761135022, ALWSD.2016.026, and EP/R024537/1), the National Natural Science Foundation of China (grant nos. 41501116, 41401413), the Research Foundation Flanders (FWO PhD grant no. L.S., 11S9614N and FWO project grant no. G.0600.18N), and the US National Science Foundation (grant nos. 1237733, 1426981, 1654374, 1832221, and 1529245).
This paper was edited by Orencio Duran Vinent and reviewed by Maarten Kleinhans and two anonymous referees.
Adamowicz, S. C. and Roman, C. T.: New England salt marsh pools: a quantitative analysis of geomorphic and geographic features, Wetlands, 25, 279–288, https://doi.org/10.1672/4, 2005.
Alkemade, I. S. W.: Kwaliteitsdocument Laseraltimetrie, Projectgebied Westerschelde, Ministerie van Verkeer en Waterstaat, Rijkswaterstaat, Delft, the Netherlands, 2004.
Allen, J.: Morphodynamics of Holocene salt marshes: a review sketch from the Atlantic and Southern North Sea coasts of Europe, Quaternay Sci. Rev., 19, 1155–1231, https://doi.org/10.1016/s0277-3791(99)00034-7, 2000.
Argow, B. A. and FitzGerald, D. M.: Winter processes on northern salt marshes: evaluating the impact of in-situ peat compaction due to ice loading, Wells, ME, Estuar. Coast. Shelf Sci., 69, 360–369, https://doi.org/10.1016/j.ecss.2006.05.006, 2006.
Bakker, R. B. and Bijkerk, W.: Toelichting Bij de Geomorfologische Kartering Westerschelde 2008 op Basis van False Colour-Luchtfoto's 1:10.000, Report, Ministerie van Verkeer en Waterstaat, Rijksinstituut, Adviesdienst Geo-Informatie & ICT, Den Haag, Delft, the Netherlands, 2009.
Balke, T., Stock, M., Jensen, K., Bouma, T. J., and Kleyer, M.: A global analysis of the seaward salt marsh extent: The importance of tidal range, Water Resour. Res., 52, 3775–3786, https://doi.org/10.1002/2015WR018318, 2016.
Barbier, E. B., Koch, E. W., Silliman, B. R., Hacker, S. D., Wolanski, E., Primavera, J., Granek, E. F., Polasky, S., Aswani, S., Cramer, L. A., Stoms, D. M., Kennedy, C. J., Bael, D., Kappel, C. V., Perillo, G. M. E., and Reed, D. J.: Coastal ecosystem-based management with nonlinear ecological functions and values, Science, 319, 321–323, https://doi.org/10.1126/science.1150349, 2008.
Barbier, E. B., Hacker, S. D., Kennedy, C., Koch, E. W., Stier, A. C., and Silliman, B. R.: The value of estuarine and coastal ecosystem services, Ecol. Monogr., 81, 169–193, https://doi.org/10.1890/10-1510.1, 2011.
Bartholdy, J.: Salt marsh sedimentation, in: Principles of Tidal Sedimentology, edited by: Davis, R. A. and Dalrymple, R. W., Springer, Dordrecht, the Netherlands, 151–185, 2012.
Baumann, R. H., Day, J. W., and Miller, C. A.: Mississippi deltaic wetland survival: sedimentation versus coastal submergence, Science, 224, 1093–1095, https://doi.org/10.1126/science.224.4653.1093, 1984.
Belluco, E., Camuffo, M., Ferrari, S., Modenese, L., Silvestri, S., Marani, A., and Marani, M.: Mapping salt-marsh vegetation by multispectral and hyperspectral remote sensing, Remote Sens. Environ., 105, 54–67, https://doi.org/10.1016/j.rse.2006.06.006, 2006.
Brückner, M. Z. M., Schwarz, C., van Dijk, W. M., van Oorschot, M., Douma, H., and Kleinhans, M. G.: Salt marsh establishment and eco-engineering effects in dynamic estuaries determined by species growth and mortality, J. Geophys. Res.-Earth, 124, 2962–2986, https://doi.org/10.1029/2019JF005092, 2019.
Carbognin, L., Teatini, P., and Tosi, L.: Eustacy and land subsidence in the Venice Lagoon at the beginning of the new millennium, J. Mar. Syst., 51, 345–353, https://doi.org/10.1016/j.jmarsys.2004.05.021, 2004.
Carniello, L., Defina, A., and D'Alpaos, L.: Morphological evolution of the Venice Lagoon: evidence from the past and trend for the future, J. Geophys. Res., 114, F04002, https://doi.org/10.1029/2008jf001157, 2009.
Christiansen, T., Wiberg, P. L., and Milligan, T. G.: Flow and sediment transport on a tidal salt marsh surface, Estuar. Coast. Shelf Sci., 50, 315–331, https://doi.org/10.1006/ecss.2000.0548, 2000.
Covi, M. P. and Kneib, R. T.: Intertidal distribution, population dynamics and production of the amphipod Uhlorchestia spartinophila in a Georgia, USA, salt marsh, Mar. Biol., 121, 447–455, https://doi.org/10.1007/bf00349453, 1995.
D'Alpaos, A.: The mutual influence of biotic and abiotic components on the long-term ecomorphodynamic evolution of salt-marsh ecosystems, Geomorphology, 126, 269–278, https://doi.org/10.1016/j.geomorph.2010.04.027, 2011.
D'Alpaos, A. and Marani, M.: Reading the signatures of biologic–geomorphic feedbacks in salt-marsh landscapes, Adv. Water Resour., 93, 265–275, https://doi.org/10.1016/j.advwatres.2015.09.004, 2016.
D'Alpaos, A., Lanzoni, S., Marani, M., and Rinaldo, A.: Landscape evolution in tidal embayments: modeling the interplay of erosion, sedimentation, and vegetation dynamics, J. Geophys. Res., 112, F01008, https://doi.org/10.1029/2006jf000537, 2007.
D'Alpaos, A., Lanzoni, S., Marani, M., and Rinaldo, A.: On the tidal prism–channel area relations, J. Geophys. Res., 115, F01003, https://doi.org/10.1029/2008jf001243, 2010.
D'Alpaos, A., Mudd, S. M., and Carniello, L.: Dynamic response of marshes to perturbations in suspended sediment concentrations and rates of relative sea level rise, J. Geophys. Res., 116, F04020, https://doi.org/10.1029/2011jf002093, 2011.
Day, J. W., Rybczyk, J., Scarton, F., Rismondo, A., Are, D., and Cecconi, G.: Soil accretionary dynamics, sea-level rise and the survival of wetlands in Venice Lagoon: a field and modelling approach, Estuar. Coast. Shelf Sci., 49, 607–628, https://doi.org/10.1006/ecss.1999.0522, 1999.
Day, J. W., Britsch, L. D., Hawes, S. R., Shaffer, G. P., Reed, D. J., and Cahoon, D.: Pattern and process of land loss in the Mississippi delta: a spatial and temporal analysis of wetland habitat change, Estuaries, 23, 425–438, https://doi.org/10.2307/1353136, 2000.
Defendi, V., Kovačević, V., Arena, F., and Zaggia, L.: Estimating sediment transport from acoustic measurements in the Venice Lagoon inlets, Cont. Shelf Res., 30, 883–893, https://doi.org/10.1016/j.csr.2009.12.004, 2010.
DeLaune, R. D., Nyman, J. A., and Patrick, J. W. H.: Peat collapse, ponding and wetland loss in a rapidly submerging coastal marsh, J. Coast. Res., 10, 1021–1030, 1994.
Elschot, K., Vermeulen, A., Vandenbruwaene, W., Bakker, J. P., Bouma, T. J., Stahl, J., Castelijns, H., and Temmerman, S.: Top-down vs. bottom-up control on vegetation composition in a tidal marsh depends on scale, PLoS One, 12, e0169960, https://doi.org/10.1371/journal.pone.0169960, 2017.
Erwin, R. M., Cahoon, D. R., Prosser, D. J., Sanders, G. M., and Hensel, P.: Surface elevation dynamics in vegetated Spartina marshes versus unvegetated tidal ponds along the Mid-Atlantic Coast, USA, with implications to waterbirds, Estuar. Coasts, 29, 96–106, https://doi.org/10.1007/bf02784702, 2006.
Esselink, P., Dijkema, K. S., Sabine, R., and Geert, H.: Vertical accretion and profile changes in abandoned man-made tidal marshes in the Dollard Estuary, the Netherlands, J. Coast. Res., 14, 570–582, 1998.
Fagherazzi, S.: The ephemeral life of a salt marsh, Geology, 41, 943–944, https://doi.org/10.1130/focus082013.1, 2013.
Fagherazzi, S., Carniello, L., D'Alpaos, L., and Defina, A.: Critical bifurcation of shallow microtidal landforms in tidal flats and salt marshes, P. Natl. Acad. Sci. USA, 103, 8337–8341, https://doi.org/10.1073/pnas.0508379103, 2006.
Fagherazzi, S., Palermo, C., Rulli, M. C., Carniello, L., and Defina, A.: Wind waves in shallow microtidal basins and the dynamic equilibrium of tidal flats, J. Geophys. Res., 112, F02024, https://doi.org/10.1029/2006jf000572, 2007.
Fagherazzi, S., Kirwan, M. L., Mudd, S. M., Guntenspergen, G. R., Temmerman, S., D'Alpaos, A., van de Koppel, J., Rybczyk, J. M., Reyes, E., Craft, C., and Clough, J.: Numerical models of salt marsh evolution: ecological, geomorphic, and climatic factors, Rev. Geophys., 50, 294–295, https://doi.org/10.1029/2011rg000359, 2012.
Fagherazzi, S., Mariotti, G., Wiberg, P., and McGlathery, K.: Marsh collapse does not require sea level rise, Oceanography, 26, 70–77, https://doi.org/10.5670/oceanog.2013.47, 2013.
Friedrichs, C. T. and Perry, J. E.: Tidal Salt Marsh Morphodynamics: A Synthesis, J. Coast. Res., 27, 7–37, 2001.
Ganju, N. K., Nidzieko, N. J., and Kirwan, M. L.: Inferring tidal wetland stability from channel sediment fluxes: observations and a conceptual model, J. Geophys. Res.-Earth, 118, 2045–2058, https://doi.org/10.1002/jgrf.20143, 2013.
Gedan, K. B., Kirwan, M. L., Wolanski, E., Barbier, E. B., and Silliman, B. R.: The present and future role of coastal wetland vegetation in protecting shorelines: answering recent challenges to the paradigm, Climatic Change, 106, 7–29, https://doi.org/10.1007/s10584-010-0003-7, 2011.
Gieskes, J. M., Elwany, H., Rasmussen, L., Han, S., Rathburn, A., and Deheyn, D. D.: Salinity variations in the Venice Lagoon, Italy: results from the SIOSED project, May 2005–February 2007, Mar. Chem., 154, 77–86, https://doi.org/10.1016/j.marchem.2013.05.011, 2013.
Harshberger, J. W.: The origin and vegetation of salt marsh pools, Proc. Am. Philos. Soc., 55, 481–484, 1916.
Hu, Z., Suzuki, T., Zitman, T., Uittewaal, W., and Stive, M.: Laboratory study on wave dissipation by vegetation in combined current–wave flow, Coast. Eng., 88, 131–142, https://doi.org/10.1016/j.coastaleng.2014.02.009, 2014.
Hu, Z., van Belzen, J., van der Wal, D., Balke, T., Wang, Z. B., Stive, M., and Bouma, T. J.: Windows of opportunity for salt marsh vegetation establishment on bare tidal flats: the importance of temporal and spatial variability in hydrodynamic forcing, J. Geophys. Res.-Biogeo., 120, 1450–1469, https://doi.org/10.1002/2014jg002870, 2015a.
Hu, Z., Wang, Z. B., Zitman, T. J., Stive, M. J. F., and Bouma, T. J.: Predicting long-term and short-term tidal flat morphodynamics using a dynamic equilibrium theory, J. Geophys. Res.-Earth, 120, 1803–1823, https://doi.org/10.1002/2015jf003486, 2015b.
Hu, Z., van der Wal, D., Cai, H., van Belzen, J., and Bouma, T. J.: Dynamic equilibrium behaviour observed on two contrasting tidal flats from daily monitoring of bed-level changes, Geomorphology, 311, 114–126, https://doi.org/10.1016/j.geomorph.2018.03.025, 2018.
Huijs, S. W. E.: Geomorfologische Ontwikkeling van Het Intergetijdegebied in de Westerschelde, 1935–1989, Rapport R 95-3, Universiteit Utrecht, Utrecht, the Netherlands, 1995.
Kearney, M. S. and Rogers, A. S.: Forecasting sites of future coastal marsh loss using topographical relationships and logistic regression, Wetl. Ecol. Manage., 18, 449–461, https://doi.org/10.1007/s11273-010-9178-y, 2010.
Kearney, M. S., Grace, R. E., and Stevenson, J. C.: Marsh loss in nanticoke estuary, Chesapeake Bay, Geogr. Rev., 78, 205–220, https://doi.org/10.2307/214178, 1988.
Kearney, M. S., Rogers, A. S., Townshend, J. R. G., Rizzo, E., Stutzer, D., Stevenson, J. C., and Sundborg, K.: Landsat imagery shows decline of coastal marshes in Chesapeake and Delaware Bays, Eos Trans. Am. Geophys. Union, 83, 173–178, https://doi.org/10.1029/2002eo000112, 2002.
Kendrot, S. R.: Restoration through eradication: protecting Chesapeake Bay marshlands from invasive nutria (Myocastor coypus), in: Island Invasives: Eradication and Management, in: Proceedings of the International Conference on Island Invasives (Occasional Papers of the IUCN Species Survival Commission Occasional Papers of the IUCN Species Survival Commission), edited by: Veitch, C. R., Clout, M. N., and Towns, D. R., IUCN, Gland, Switzerland, 313–319, 2011.
Kirwan, M. L. and Guntenspergen, G. R.: Influence of tidal range on the stability of coastal marshland, J. Geophys. Res.-Earth, 115, F02009, https://doi.org/10.1029/2009jf001400, 2010.
Kirwan, M. L. and Guntenspergen, G. R.: Feedbacks between inundation, root production, and shoot growth in a rapidly submerging brackish marsh, J. Ecol., 100, 764–770, https://doi.org/10.1111/j.1365-2745.2012.01957.x, 2012.
Kirwan, M. L. and Guntenspergen, G. R.: Response of plant productivity to experimental flooding in a stable and a submerging marsh, Ecosystems, 18, 903–913, https://doi.org/10.1007/s10021-015-9870-0, 2015.
Kirwan, M. L. and Megonigal, J. P.: Tidal wetland stability in the face of human impacts and sea-level rise, Nature, 504, 53–60, https://doi.org/10.1038/nature12856, 2013.
Kirwan, M. L. and Murray, A. B.: A coupled geomorphic and ecological model of tidal marsh evolution, P. Natl. Acad. Sci. USA, 104, 6118–6122, https://doi.org/10.1073/pnas.0700958104, 2007.
Kirwan, M. L., Murray, A. B., and Boyd, W. S.: Temporary vegetation disturbance as an explanation for permanent loss of tidal wetlands, Geophys. Res. Lett., 35, L05403, https://doi.org/10.1029/2007gl032681, 2008.
Kirwan, M. L., Guntenspergen, G. R., D'Alpaos, A., Morris, J. T., Mudd, S. M., and Temmerman, S.: Limits on the adaptability of coastal marshes to rising sea level, Geophys. Res. Lett., 37, 58–94, https://doi.org/10.1029/2010gl045489, 2010.
Kirwan, M. L., Murray, A. B., Donnelly, J. P., and Corbett, D. R.: Rapid wetland expansion during European settlement and its implication for marsh survival under modern sediment delivery rates, Geology, 39, 507–510, https://doi.org/10.1130/g31789.1, 2011.
Leonard, L. A.: Controls of sediment transport and deposition in an incised mainland marsh basin, Southeastern North Carolina, Wetlands, 17, 263–274, https://doi.org/10.1007/bf03161414, 1997.
Marani, M., Belluco, E., D'Alpaos, A., Defina, A., Lanzoni, S., and Rinaldo, A.: On the drainage density of tidal networks, Water Resour. Res., 39, 5029–5035, https://doi.org/10.1029/2001wr001051, 2003.
Marani, M., Silvestri, S., Belluco, E., Ursino, N., Comerlati, A., Tosatto, O., and Putti, M.: Spatial organization and ecohydrological interactions in oxygen-limited vegetation ecosystems, Water Resour. Res., 42, 387–403, https://doi.org/10.1029/2005wr004582, 2006.
Marani, M., D'Alpaos, A., Lanzoni, S., Carniello, L., and Rinaldo, A.: Biologically-controlled multiple equilibria of tidal landforms and the fate of the Venice Lagoon, Geophys. Res. Lett., 34, 224–238, https://doi.org/10.1029/2007gl030178, 2007.
Marani, M., D'Alpaos, A., Lanzoni, S., Carniello, L., and Rinaldo, A.: The importance of being coupled: stable states and catastrophic shifts in tidal biomorphodynamics, J. Geophys. Res., 115, F04004, https://doi.org/10.1029/2009jf001600, 2010.
Mariotti, G.: Revisiting salt marsh resilience to sea level rise: are ponds responsible for permanent land loss?, J. Geophys. Res.-Earth, 121, 1391–1407, https://doi.org/10.1002/2016jf003900, 2016.
Mariotti, G. and Fagherazzi, S.: A numerical model for the coupled long-term evolution of salt marshes and tidal flats, J. Geophys. Res., 115, F01004, https://doi.org/10.1029/2009jf001326, 2010.
Mariotti, G. and Fagherazzi, S.: Critical width of tidal flats triggers marsh collapse in the absence of sea-level rise, P. Natl. Acad. Sci. USA, 110, 5353–5356, https://doi.org/10.1073/pnas.1219600110, 2013.
McGlathery, K., Reidenbach, M., D'Odorico, P., Fagherazzi, S., Pace, M., and Porter, J.: Nonlinear dynamics and alternative stable states in shallow coastal systems, Oceanography, 26, 220–231, https://doi.org/10.5670/oceanog.2013.66, 2013.
McLeod, E., Chmura, G. L., Bouillon, S., Salm, R., Björk, M., Duarte, C. M., Lovelock, C. E., Schlesinger, W. H., and Silliman, B. R.: A blueprint for blue carbon: toward an improved understanding of the role of vegetated coastal habitats in sequestering CO2, Front. Ecol. Environ., 9, 552–560, https://doi.org/10.1890/110004, 2011.
Miller, W. R. and Egler, F. E.: Vegetation of the wequetequock-pawcatuck tidal-marshes, Connecticut, Ecol. Monogr., 20, 143–172, https://doi.org/10.2307/1943548, 1950.
Millette, T. L., Argow, B. A., Marcano, E., Hayward, C., Hopkinson, C. S., and Valentine, V.: Salt marsh geomorphological analysesviaintegration of multitemporal multispectral remote sensing with LIDAR and GIS, J. Coast. Res., 265, 809–816, https://doi.org/10.2112/jcoastres-d-09-00101.1, 2010.
Moffett, K., Nardin, W., Silvestri, S., Wang, C., and Temmerman, S.: Multiple stable states and catastrophic shifts in coastal wetlands: progress, challenges, and opportunities in validating theory using remote sensing and other methods, Remote Sens., 7, 10184–10226, https://doi.org/10.3390/rs70810184, 2015.
Morton, R. A., Tiling, G., and Ferina, N. F.: Causes of hot-spot wetland loss in the Mississippi delta plain, Environ. Geosci., 10, 71–80, https://doi.org/10.1306/eg100202007, 2003.
Moskalski, S. M. and Sommerfield, C. K.: Suspended sediment deposition and trapping efficiency in a Delaware salt marsh, Geomorphology, 139–140, 195–204, https://doi.org/10.1016/j.geomorph.2011.10.018, 2012.
Mudd, S. M., D'Alpaos, A., and Morris, J. T.: How does vegetation affect sedimentation on tidal marshes? Investigating particle capture and hydrodynamic controls on biologically mediated sedimentation, J. Geophys. Res., 115, F03029, https://doi.org/10.1029/2009jf001566, 2010.
Neumeier, U. R. S. and Amos, C. L.: The influence of vegetation on turbulence and flow velocities in European salt-marshes, Sedimentology, 53, 259–277, https://doi.org/10.1111/j.1365-3091.2006.00772.x, 2006.
Nyman, J. A., Walters, R. J., Delaune, R. D., and Patrick, W. H.: Marsh vertical accretion via vegetative growth, Estuar. Coast. Shelf Sci., 69, 370–380, https://doi.org/10.1016/j.ecss.2006.05.041, 2006.
OCM Partners: 2003 Maryland Department of Natural Resources LiDAR: Dorchester, Somerset, Talbot, and Wicomico Counties, with portions of Caroline, Kent and Queen Anne's Counties, available at: https://www.fisheries.noaa.gov/inport/item/49781, last access: 6 February 2021.
Ortiz, A. C., Roy, S., and Edmonds, D. A.: Land loss by pond expansion on the Mississippi river delta plain, Geophys. Res. Lett., 44, 3635–3642, https://doi.org/10.1002/2017gl073079, 2017.
Pendleton, E. and Stevenson, J.: Investigations of Marsh Losses at Blackwater Refuge: Final Report, University of Maryland Center for Environmental and Estuarine Studies, Cambridge, MA, 1983.
Penland, S., Wayne, L., Britsch, L. D., Williams, S. J., Beall, A. D., and Butterworth, V. C.: Geomorphic Classification of Coastal Land Loss between 1932 and 1990 in the Mississippi River Delta Plain, Southeastern Louisiana, USGS Open File Report 00-417, Coastal Marine Geology Program, US Geological Survey, Woods Hole, MA, 2000.
planetek italia: ikonos, available at: https://www.planetek.it/prodotti/tutti_i_prodotti/ikonos, last access: 6 February 2021.
R Core Team: A Language and Environment for Statistical Computing R, Vienna, Austria, 2016.
Redfield, A. C.: Development of a New England salt marsh, Ecol. Monogr., 42, 201–237, https://doi.org/10.2307/1942263, 1972.
Reed, D. J.: Sediment dynamics and deposition in a retreating coastal salt marsh, Estuar. Coast. Shelf Sci., 26, 67–79, https://doi.org/10.1016/0272-7714(88)90012-1, 1988.
Reed, D. J., Spencer, T., Murray, A. L., French, J. R., and Leonard, L.: Marsh surface sediment deposition and the role of tidal creeks: implications for created and managed coastal marshes, J. Coast. Conserv., 5, 81–90, https://doi.org/10.1007/bf02802742, 1999.
Reitsma, J. M.: Toelichting Bij de Vegetatiekartering Westerschelde 2004 op Basis Vanfalse Colour-Luchtfoto's , Report, Ministerie van Verkeer en Waterstaat, Rijksinstituut, Adviesdienst Geo-Informatie & ICT, Den Haag, Delft, the Netherlands, 2006.
Rijkswaterstaat: Open data Rijkswaterstaat, available at: https://www.rijkswaterstaat.nl/zakelijk/open-data, last access: 6 February 2021.
Rinaldo, A., Fagherazzi, S., Lanzoni, S., Marani, M., and Dietrich, W. E.: Tidal networks: 2. Watershed delineation and comparative network morphology, Water Resour. Res., 35, 3905–3917, https://doi.org/10.1029/1999wr900237, 1999a.
Rinaldo, A., Fagherazzi, S., Lanzoni, S., Marani, M., and Dietrich, W. E.: Tidal networks: 3. Landscape-forming discharges and studies in empirical geomorphic relationships, Water Resour. Res., 35, 3919–3929, https://doi.org/10.1029/1999wr900238, 1999b.
Roner, M., D'Alpaos, A., Ghinassi, M., Marani, M., Silvestri, S., Franceschinis, E., and Realdon, N.: Spatial variation of salt-marsh organic and inorganic deposition and organic carbon accumulation: inferences from the Venice Lagoon, Italy, Adv. Water Resour., 93, 276–287, https://doi.org/10.1016/j.advwatres.2015.11.011, 2016.
Sarretta, A., Pillon, S., Molinaroli, E., Guerzoni, S., and Fontolan, G.: Sediment budget in the Lagoon of Venice, Italy, Cont. Shelf Res., 30, 934–949, https://doi.org/10.1016/j.csr.2009.07.002, 2010.
Scheffer, M. and Carpenter, S. R.: Catastrophic regime shifts in ecosystems: linking theory to observation, Trends Ecol. Evol., 18, 648–656, https://doi.org/10.1016/j.tree.2003.09.002, 2003.
Scheffer, M., Carpenter, S., Foley, J. A., Folke, C., and Walker, B.: Catastrophic shifts in ecosystems, Nature, 413, 591–596, https://doi.org/10.1038/35098000, 2001.
Schepers, L., Kirwan, M., Guntenspergen, G., and Temmerman, S.: Spatio-temporal development of vegetation die-off in a submerging coastal marsh, Limnol. Oceanogr., 62, 137–150, https://doi.org/10.1002/lno.10381, 2017.
Silliman, B. R.: Drought, snails, and large-scale die-off of Southern U.S. salt marshes, Science, 310, 1803–1806, https://doi.org/10.1126/science.1118229, 2005.
Silvestri, S., Defina, A., and Marani, M.: Tidal regime, salinity and salt marsh plant zonation, Estuar. Coast. Shelf Sci., 62, 119–130, https://doi.org/10.1016/j.ecss.2004.08.010, 2005.
Stevenson, J. C., Kearney, M. S., and Pendleton, E. C.: Sedimentation and erosion in a Chesapeake Bay brackish marsh system, Mar. Geol., 67, 213–235, https://doi.org/10.1016/0025-3227(85)90093-3, 1985.
Temmerman, S. and Kirwan, M. L.: Building land with a rising sea, Science, 349, 588–589, https://doi.org/10.1126/science.aac8312, 2015.
Temmerman, S., Govers, G., Meire, P., and Wartel, S.: Modelling long-term tidal marsh growth under changing tidal conditions and suspended sediment concentrations, Scheldt estuary, Belgium, Mar. Geol., 193, 151–169, https://doi.org/10.1016/s0025-3227(02)00642-4, 2003a.
Temmerman, S., Govers, G., Wartel, S., and Meire, P.: Spatial and temporal factors controlling short-term sedimentation in a salt and freshwater tidal marsh, Scheldt estuary, Belgium, SW Netherlands, Earth Surf. Proc. Land., 28, 739–755, https://doi.org/10.1002/esp.495, 2003b.
Temmerman, S., Govers, G., Meire, P., and Wartel, S.: Simulating the long-term development of levee–basin topography on tidal marshes, Geomorphology, 63, 39–55, https://doi.org/10.1016/j.geomorph.2004.03.004, 2004.
Temmerman, S., Moonen, P., Schoelynck, J., Govers, G., and Bouma, T. J.: Impact of vegetation die-off on spatial flow patterns over a tidal marsh, Geophys. Res. Lett., 39, L03406, https://doi.org/10.1029/2011gl050502, 2012.
Temmerman, S., Meire, P., Bouma, T. J., Herman, P. M. J., Ysebaert, T., and De Vriend, H. J.: Ecosystem-based coastal defence in the face of global change, Nature, 504, 79–83, https://doi.org/10.1038/nature12859, 2013.
Tommasini, L., Carniello, L., Ghinassi, M., Roner, M., and D'Alpaos, A.: Changes in the wind-wave field and related salt-marsh lateral erosion: inferences from the evolution of the Venice Lagoon in the last four centuries, Earth Surf. Proc. Land., 44, 1633–1646, https://doi.org/10.1002/esp.4599, 2019.
Turner, R. E. and Rao, Y. S.: Relationships between wetland fragmentation and recent hydrologic changes in a deltaic coast, Estuaries, 13, 272–281, https://doi.org/10.2307/1351918, 1990.
Ursino, N., Silvestri, S., and Marani, M.: Subsurface flow and vegetation patterns in tidal environments, Water Resour. Res., 40, 191–201, https://doi.org/10.1029/2003wr002702, 2004.
USGS: EarthExplorer, available at: https://earthexplorer.usgs.gov/, last access: 6 February 2021.
van Belzen, J., van de Koppel, J., Kirwan, M. L., van der Wal, D., Herman, P. M. J., Dakos, V., Kéfi, S., Scheffer, M., Guntenspergen, G. R., and Bouma, T. J.: Vegetation recovery in tidal marshes reveals critical slowing down under increased inundation, Nat. Commun., 8, 15811, https://doi.org/10.1038/ncomms15811, 2017.
van Damme, S., Struyf, E., Maris, T., Ysebaert, T., Dehairs, F., Tackx, M., Heip, C., and Meire, P.: Spatial and temporal patterns of water quality along the estuarine salinity gradient of the Scheldt estuary (Belgium and The Netherlands): results of an integrated monitoring approach, Hydrobiologia, 540, 29–45, https://doi.org/10.1007/s10750-004-7102-2, 2005.
Vandenbruwaene, W., Temmerman, S., Bouma, T. J., Klaassen, P. C., de Vries, M. B., Callaghan, D. P., van Steeg, P., Dekker, F., van Duren, L. A., Martini, E., Balke, T., Biermans, G., Schoelynck, J., and Meire, P.: Flow interaction with dynamic vegetation patches: implications for biogeomorphic evolution of a tidal landscape, J. Geophys. Res.-Earth, 116, 155–170, https://doi.org/10.1029/2010jf001788, 2011.
Vandenbruwaene, W., Bouma, T. J., Meire, P., and Temmerman, S.: Bio-geomorphic effects on tidal channel evolution: impact of vegetation establishment and tidal prism change, Earth Surf. Proc. Land., 38, 122–132, https://doi.org/10.1002/esp.3265, 2013.
van der Pluijm, A. M. and de Jong, D. J.: Historisch Overzicht Schorareaal in Zuid-West Nederland, Report Werkdocument RIKZ/OS-98.860 x, Rijkswaterstaat –Rijksinstituut voor Kust en Zee, Utrecht, the Netherlands, 1998.
van Wesenbeeck, B. K., van de Koppel, J., Herman, P. M. J., Bertness, M. D., van der Wal, D., Bakker, J. P., and Bouma, T. J.: Potential for sudden shifts in transient systems: distinguishing between local and landscape-scale processes, Ecosystems, 11, 1133–1141, https://doi.org/10.1007/s10021-008-9184-6, 2008.
Venier, C., D'Alpaos, A., and Marani, M.: Evaluation of sediment properties using wind and turbidity observations in the shallow tidal areas of the Venice Lagoon, J. Geophys. Res.-Earth, 119, 1604–1616, https://doi.org/10.1002/2013jf003019, 2014.
Wamsley, T. V., Cialone, M. A., Smith, J. M., Atkinson, J. H., and Rosati, J. D.: The potential of wetlands in reducing storm surge, Ocean Eng., 37, 59–68, https://doi.org/10.1016/j.oceaneng.2009.07.018, 2010.
Wang, C. and Temmerman, S.: Does biogeomorphic feedback lead to abrupt shifts between alternative landscape states? An empirical study on intertidal flats and marshes, J. Geophys. Res.-Earth, 118, 229–240, https://doi.org/10.1029/2012jf002474, 2013.
Wang, C., Menenti, M., Stoll, M. P., Feola, A., Belluco, E., and Marani, M.: Separation of ground and low vegetation signatures in LiDAR measurements of salt-marsh environments, IEEE T. Geosci. Remote, 47, 2014–2023, https://doi.org/10.1109/tgrs.2008.2010490, 2009.
Wilson, C. A., Hughes, Z. J., FitzGerald, D. M., Hopkinson, C. S., Valentine, V., and Kolker, A. S.: Saltmarsh pool and tidal creek morphodynamics: dynamic equilibrium of northern latitude saltmarshes?, Geomorphology, 213, 99–115, https://doi.org/10.1016/j.geomorph.2014.01.002, 2014.
Wilson, K. R., Kelley, J. T., Croitoru, A., Dionne, M., Belknap, D. F., and Steneck, R.: Stratigraphic and ecophysical characterizations of salt pools: dynamic landforms of the webhannet salt marsh, Wells, ME, USA, Estuar. Coasts, 32, 855–870, https://doi.org/10.1007/s12237-009-9203-7, 2009.
Wilson, K. R., Kelley, J. T., Tanner, B. R., and Belknap, D. F.: Probing the origins and stratigraphic signature of salt pools from north-temperate marshes in Maine, U.S.A., J. Coast. Res., 26, 1007–1026, https://doi.org/10.2112/jcoastres-d-10-00007.1, 2010.
Yang, S. L., Shi, B. W., Bouma, T. J., Ysebaert, T., and Luo, X. X.: Wave attenuation at a salt marsh margin: a case study of an exposed coast on the yangtze estuary, Estuar. Coasts, 35, 169–182, https://doi.org/10.1007/s12237-011-9424-4, 2012.
Zaggia, L. and Ferla, M.: Studies on water and suspended sediment transport at the Venice Lagoon inlets, in: Proceedings of the Fifth International Symposium WAVES, 3–7 July 2005, Madrid, Spain, 1–10, 2005.
Zirino, A., Elwany, H., Neira, C., Maicu, F., Mendoza, G., and Levin, L. A.: Salinity and its variability in the Lagoon of Venice, 2000–2009, Adv. Oceanogr. Limnol., 5, 41–59, https://doi.org/10.4081/aiol.2014.5350, 2014.