the Creative Commons Attribution 4.0 License.
the Creative Commons Attribution 4.0 License.
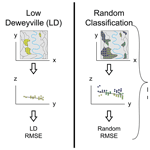
A multi-proxy assessment of terrace formation in the lower Trinity River valley, Texas
Hima J. Hassenruck-Gudipati
Thaddeus Ellis
Timothy A. Goudge
David Mohrig
A proposed null hypothesis for fluvial terrace formation is that internally generated or autogenic processes, such as lateral migration and river-bend cutoff, produce variabilities in channel incision that lead to the abandonment of floodplain segments as terraces. Alternatively, fluvial terraces have the potential to record past environmental changes from external forcings that include temporal changes in sea level and hydroclimate. Terraces in the Trinity River valley have been previously characterized as Deweyville groups and interpreted to record episodic cut and fill during late Pleistocene sea level variations. Our study uses high-resolution topography of a bare-earth digital elevation model derived from airborne lidar surveys along ∼ 88 linear kilometers of the modern river valley. We measure both differences in terrace elevations and widths of paleo-channels preserved on these terraces in order to have two independent constraints on terrace formation mechanisms. For 52 distinct terraces, we quantify whether terrace elevations fit distinct planes – expected for allogenic terrace formation tied to punctuated sea level and/or hydroclimate change – by comparing variability in a grouped set of Deweyville terrace elevations against variability associated with randomly selected terrace sets. Results show Deweyville groups record an initial valley floor abandoning driven by allogenic forcing, which transitions into autogenic forcing for the formation of younger terraces. For these different terrace sets, the slope amongst different terraces stays constant. For 79 paleo-channel segments preserved on these terraces, we connected observed changes in paleo-channel widths to estimates for river paleo-hydrology over time. Our measurements suggest the discharge of the Trinity River increased systematically by a factor of ∼ 2 during the late Pleistocene. Despite this evidence of increased discharge, the similar down-valley slopes between terrace sets indicate that there were likely no increases in sediment-to-water discharge ratios that could be linked to allogenic terrace formation. This is consistent with our elevation clustering analysis that suggests younger terraces are indistinguishable in their elevation variance from autogenic terrace formation mechanisms, even if the changing paleo-channel dimensions might, viewed in isolation, provide a mechanism for allogenic terrace formation. Methods introduced here combine river-reach-scale observations of terrace sets and paleo-hydrology with local observations of terraces and paleo-channels to show how interpretations of allogenic versus autogenic terrace formation can be evaluated within a single river system.
- Article
(8300 KB) - Full-text XML
-
Supplement
(30168 KB) - BibTeX
- EndNote
River valleys commonly contain fluvial terraces representing segments of older floodplain that are now located at elevations distinctly above the modern floodplain. These terraces sometimes preserve paleo-channels or remnant river-channel segments. For exceptionally preserved features, channel widths, depths, bend amplitudes and wavelengths, and grain size record a signal of past river hydrology. Terrace formation requires net river incision that can be allogenically driven by tectonic uplift, sea level fall, and/or modifications to water and sediment discharge via climate change or land use change, including dam construction (Bull, 1990; Hancock and Anderson, 2002; Mackey et al., 2011; Pazzaglia, 2013; Womack and Schumm, 1977). What is more controversial is the character of the trigger that leads to the relatively discrete transfer of a section of active floodplain or valley floor into an inactive terrace or set of terraces elevated above flood height. In particular, can terraces formed by a punctuated sea level fall, tectonic uplift, or sediment-to-water flux change be accurately separated from terraces formed by lateral migration and incision connected with the autogenic processes of river channel migration and channel-bend cutoff? Here we use attributes of terraces and their preserved paleo-channels in the coastal Trinity River valley in order to evaluate the likelihood of allogenic versus autogenic triggers driving terrace formation for previously established groups of Deweyville terraces (Bernard, 1950; Blum et al., 1995). Understanding how these terraces were most likely formed will help to constrain interpretations of the input signals for downstream deltaic deposits, which are recognized to embed both allogenic and autogenic signals (Guerit et al., 2020).
Commonly invoked allogenic triggers connected with terrace formation are (1) punctuated decreases in sediment-to-water flux that are assumed to embed a signal of regional climate change and (2) punctuated base level fall controlled by either sea level fall or tectonic uplift, all of which can drive periods of increased vertical incision along an extended length of river channel (Blum et al., 1995; Blum and Törnqvist, 2000; Bull, 1990; Daley and Cohen, 2018; Hancock and Anderson, 2002; Merritts et al., 1994; Pazzaglia, 2013; Pazzaglia and Gardner, 1993; Rodriguez et al., 2005; Wegmann and Pazzaglia, 2002). These focused periods of downcutting are interpreted to produce a spatially extensive terrace (or set of terraces) that preserves a fraction of the active fluvial surface and its river channel at the time of the terrace-forming event (Bull, 1990; Molnar et al., 1994; Pazzaglia, 2013; Pazzaglia et al., 1998). One expected morphology for terraces formed by allogenic triggers is extensive terraces flanking both sides of the river at a similar elevation, which would be expected during synchronous river incision. However, it is important to realize that the extent and pairing of these terraces can be substantially modified during ongoing valley incision and that unequal channel migration during relatively slow incision rates can produce similar characteristics (Limaye and Lamb, 2016; Malatesta et al., 2017).
Both theory (Parker et al., 1998a; Wickert and Schildgen, 2019) and experiments (Tofelde et al., 2019; Whipple et al., 1998) have shown how the long profile of a fluvial valley is set by the ratio of sediment-to-water discharge. Decreases in water-to-sediment flux led to slope increases via alluviation. Conversely, increases in water-to-sediment flux produce lower slopes through channel incision and valley formation. An allogenic trigger for terrace formation associated with paleo-hydrology change is therefore expected to produce a long profile for older terraces that are steeper than the long profile of the younger and incising river. This reduction in slopes from older terrace sets to the modern floodplain has been observed in both natural (Poisson and Avouas, 2004) and experimental (Tofelde et al., 2019) systems. Interestingly, a change in climate that produced similar decreases or increases in both the water and sediment discharges would yield no change in the downstream slope of the system and no episode of incision to drive terrace formation. Since water and sediment discharges are strongly correlated within fluvial systems (Blom et al., 2017; Lane, 1955), it is quite possible that climate change might not provide an allogenic trigger for terrace formation. If long profiles extracted from terrace sets are parallel to the slope of the modern river, a different driver of incision must be at work. In the greater coastal zone this can be a base level drop tied to sea level fall (Tofelde et al., 2019). For this reason it is tempting to use interpreted sets of subparallel terraces as a proxy record for fluctuations in sea level through time (Blum et al., 1995; Blum and Törnqvist, 2000; Merritts et al., 1994; Rodriguez et al., 2005).
It has also been shown that terraces can form by autogenic processes that drive spatially variable incision rates under conditions of persistent, allogenically forced base level fall (Bull, 1990; Finnegan and Dietrich, 2011; Limaye and Lamb, 2014; Merritts et al., 1994; Muto and Steel, 2004; Strong and Paola, 2006). Autogenic terraces can be produced by channel narrowing (Lewin and Macklin, 2003; Muto and Steel, 2004; Strong and Paola, 2006) and river-bend cutoff (Erkens et al., 2009), both of which can increase bed incision rates via upstream-propagating knickpoints (Finnegan and Dietrich, 2011). Processes that lead to terraces that have autogenic characteristics include local variations in channel dynamics, channel bed slope, and sediment contribution from tributaries (Erkens et al., 2009; Lewin and Macklin, 2003; Womack and Schumm, 1977). In particular, river bend cutoff can locally increase the channel slope, driving channel-bed incision that transitions a segment of floodplain into a terrace (Erkens et al., 2009; Finnegan and Dietrich, 2011). This autogenic trigger produces terrace heights consistent with elevation drops associated with bend cutoffs (Finnegan and Dietrich, 2011). An additional autogenic process that can trigger terrace formation is variable rates of lateral channel migration during persistent base level fall (Lewin and Macklin, 2003; Limaye and Lamb, 2016). Both unsteady lateral migration and bend cutoff preferentially generate terraces that host only a small number of paleo-channel bends (Finnegan and Dietrich, 2011).
Here we present a study of three previously classified sets of fluvial terraces that make up the Deweyville allostratigraphic units of the lower Trinity River valley and that have been previously described as occurring at three distinct elevation trends (Bernard, 1950; Blum et al., 1995; Heinrich et al., 2020; Young et al., 2012). These terraces have been interpreted as forming in response to allogenic triggers that include Pleistocene sea level fluctuations and climate-controlled changes in water-to-sediment discharge (Anderson et al., 2016; Blum et al., 2013, 1995; Blum and Aslan, 2006; Rodriguez et al., 2005; Saucier and Fleetwood, 1970). We analyze whether these purported allogenic triggers can be distinguished from a null hypothesis that terraces were formed by autogenic processes during long-term valley incision associated with persistent sea level fall during the Last Glacial Period (from the end of the Eemian to the Last Glacial Maximum, LGM). To do this we implement a multi-proxy approach that (1) compares variability in terrace elevations for each classified set against elevation variability for randomly selected terraces, (2) evaluates temporal changes in paleo-hydrology as defined by segments of paleo-channels preserved on terrace surfaces, and (3) relates paleo-slopes defined by the terrace sets to the long profile of the modern Trinity River. Our analysis reveals that the upper set of terraces is most likely the product of an allogenic trigger, while the lowest set of terraces is most likely the product of autogenic processes. The formational driver for the third (intermediate) set of terraces is equivocal. This result documents how the study of terraces can be employed to substantially refine paleo-environmental interpretations that are generated using these preserved fragments of relict landscapes.
The Trinity River has the largest drainage basin contained entirely within the state of Texas, with an area of over 46 000 km2. It flows from northwest of Dallas, Texas, to Trinity Bay, where it empties into the Gulf of Mexico. Our study area is an ∼ 88 linear kilometer stretch of the lowermost Trinity River valley from just north of Romayor, Texas, to just north of Wallisville, Texas (Fig. 1). Prone to flooding, the Trinity River has a median peak annual discharge of 1679 m3 s−1 at Romayor, Texas (USGS 08066500), and 1484 m3 s−1 at Liberty, Texas (USGS 08067000), for the 2000–2020 hydrograph (U.S. Geological Survey, 2020a, b).
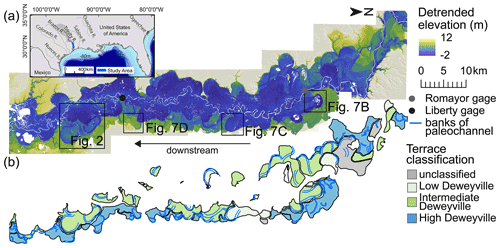
Figure 1(a) The 2011 bare-earth digital elevation model (DEM) from airborne lidar with (b) terrace and paleo-channel outlines of the Trinity River, Texas, valley. (a) The lidar DEM has been detrended using the modern valley slope to emphasize local elevation variability. The black boxes mark the extent of Figs. 2 and 7b–d. USGS gauge stations at Romayor and Liberty are marked in grey and black, respectively. The downstream extent of the data is ∼ 10 linear kilometers upstream of the river outlet into the Trinity Bay of the Galveston Bay. (b) Terraces are preferentially distributed on the east of the valley.
The Trinity River has been subject to climate and sea level variations throughout the Quaternary (Anderson et al., 2014; Galloway et al., 2000; Simms et al., 2007); however, the river catchment has never been glaciated and is interpreted to have maintained an approximately constant drainage area over this time (Hidy et al., 2014). The lower Trinity River valley is incised into the Beaumont and Lissie formations of Middle to Late Pleistocene age (Baker, 1995). Within the valley, Deweyville terraces are post-Beaumont in age and formed prior to the Holocene (Fig. 2a–b). Age-equivalent terraces with preserved segments of large paleo-channels are also found in alluvial valleys ranging from Mexico to South Carolina and are often classified as belonging to the same Deweyville bounding surface and allostratigraphic unit. Traditionally, the formation of Deweyville terraces has been interpreted as the product of high-frequency Pleistocene sea level cycles (Anderson et al., 2016; Bernard, 1950; Blum et al., 1995) with distinct episodes of incision and subsequent valley deposition (Blum et al., 2013; Blum and Aslan, 2006). The history of climatic variation, lack of glaciation, and superb preservation of late Pleistocene terraces make the lower Trinity River valley an ideal location to study terrace formation and to ask what processes these geomorphic features record.
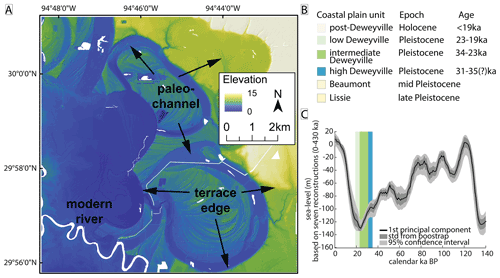
Figure 2(a) Morphological features of the Trinity River valley. Several terraces are preserved at different elevations, with the black arrows marking the edges of the terraces. The labeled paleo-channel has a width that is ∼ 2 times the modern river channel width. (b) Regional stratigraphic framework (Garvin, 2008; Blum et al., 2013). (c) Global sea level from seven reconstructions based on Spratt and Lisiecki (2016) and Deweyville terrace ages from (b) in light green, green, and blue.
The Deweyville terraces are associated with bounding surfaces of three allostratigraphic units, and can be grouped into three sets of terraces: high, intermediate, and low (Bernard, 1950; Blum et al., 1995; Young et al., 2012). Sea-level rise during the Holocene has induced valley-floor sedimentation that has partially buried the low-terrace bounding surface (Blum et al., 1995; Blum and Aslan, 2006). Age control for terraces in the lower Trinity River valley is limited to eight dates using optically stimulated luminescence (OSL) (Garvin, 2008). Based on these data, Garvin (2008) reports an OSL age of 35–31 ka for channel activity on high Deweyville terraces (N=1), 34–23 ka for intermediate Deweyville terraces (N=4), and 23–19 ka for low Deweyville terraces (N=3). With only a single OSL date from the high Deweyville terraces, these features could be as old as 60–65 ka based on existing stratigraphic frameworks (Blum et al., 2013).
The global sea level curve shows an overall range of ∼ 33 m between 35 and 19 ka (Fig. 2c, Spratt and Lisiecki, 2016). The Pleistocene sea level curve for the Gulf of Mexico during the period of Deweyville terrace formation shows high-frequency variability superimposed on a longer-term net sea level fall (Anderson et al., 2016; Simms et al., 2007). Between 35 and 19 ka, short-term rises and falls in sea level are estimated to have been as large as 20 and 60 m, respectively (Anderson et al., 2016). Deweyville bounding surfaces have been interpreted to represent three discrete sets of terraces formed during distinct oscillations in sea level (Anderson et al., 2016; Bernard, 1950; Blum et al., 1995; Morton et al., 1996; Rodriguez et al., 2005; Thomas and Anderson, 1994). The three sets of terraces also have been interpreted as recording episodes of relative sea level stasis with extensive lateral migration of the river channel, separated by punctuated incision tied to accelerated sea level fall (Blum et al., 2013; Blum and Aslan, 2006). The commonality between these two interpretations is an allogenic driver for terrace formation.
Paleo-channels have long been recognized to record past hydrologic conditions and associated climatic variations (Church, 2006; Knox, 1985). Terraces of the Trinity River valley preserve segments of abandoned river channels that range in apparent widths and depths (Fig. 2). Previous researchers have interpreted increases in these paleo-channel widths and radii of curvature for paleo-channel bends as products of increases in river discharge and precipitation (Church, 2006; Knox, 1985; Saucier and Fleetwood, 1970; Sylvia and Galloway, 2006) and possible associated changes in vegetation and/or bank erodibility (Alford and Holmes, 1985; Blum et al., 1995; Saucier, 1994). Paleo-channel morphologies thus provide a record of external paleo-environmental change in the lower Trinity River valley that is independent of any signal encapsulated in terrace formation. Therefore, by using both terrace elevations and paleo-channels, we have two geomorphic proxies to compare and contrast while assessing terrace formational processes among the Deweyville bounding surfaces.
Following the proposal of Limaye and Lamb (2016), our null hypothesis for terrace formation is that punctuated incision by autogenic triggers dominate terrace development. Only after formational mechanisms internal to the system have been considered and rejected should we consider allogenic triggers for terrace formation. Our method for testing the null hypothesis acts to separate the regional expression of an allogenic driver from more localized terrace production by autogenic processes. It is based on the observation that allogenic triggers produce synchronous, regionally extensive terraces that approximately preserve surface elevations defining a single paleo-valley slope (Bull, 1990; Pazzaglia et al., 1998). It therefore follows that a group of terraces formed by a contemporaneous allogenic trigger should preserve lower variability in elevations about a best-fit plane estimating this paleo-slope than groupings of randomly selected terraces (Fig. 3a). Conversely, autogenically produced terraces preserve a multitude of elevations that we do not expect to define a contemporaneous long profile (Fig. 3b). Therefore, groupings of local autogenic terraces are expected to be indistinguishable from sets composed of randomly selected terraces.
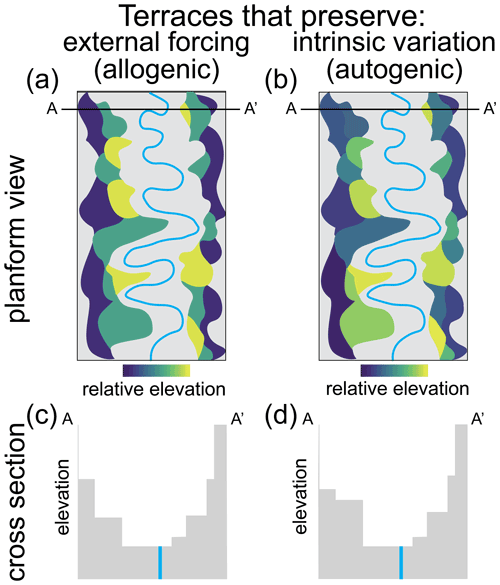
Figure 3Conceptual diagram showing distribution of terrace elevations expected in planform view (a–b) and cross-sectional view (c–d) for allogenic triggers (a, c) and autogenic triggers that form terraces (b, d). Here we show three distinct terrace sets for autogenic triggers and an undistinguishable number of terrace sets.
Our study used elevation data derived from four airborne lidar surveys collected for the Federal Emergency Management Agency (FEMA) and Texas' Strategic Mapping Program (StartMap) in 2011, 2017, and 2018 (FEMA, 2011; StartMap, 2017a, b, 2018). These four surveys were merged to produce a single bare-earth digital elevation model (DEM) with 1 m grid spacing. The horizontal accuracies of the four original lidar point clouds from 2011, 2017a, 2017b, and 2018 are 0.6 and 0.4, 0.25 and 0.29, 0.20 and 0.20, and 0.20 and 0.20 m, respectively. All data were referenced to the NAD83 horizontal datum. The vertical accuracy for the original lidar point clouds from 2011, 2017a, 2017b, and 2018 are 0.4, 0.29, 0.20, and 0.20 m, respectively, and all data were referenced to the NAVD88 vertical datum.
Individual terraces and paleo-channels were manually mapped on the merged DEM using ArcGIS. A terrace was defined as a genetically similar surface that is offset in elevation from its surrounding topography. Previously, Blum et al. (1995) mapped terraces on the Trinity River, which was extended by Garvin (2008) using a combination of satellite images and DEMs. Based on these maps, terraces were classified as high, intermediate, or low Deweyville or marked as unclassified if the surface had not been previously identified in Garvin (2008). Care was taken to only map the sections of terrace surfaces that did not appear to be modified by later fluvial processes. Elevations defining each terrace were extracted from the DEM using a 5 m grid resolution for a total of 164 520 measurements across all mapped terraces. A grid resolution lower than the DEM resolution was selected to conserve available computational resources and to speed up analyses. Mapping on the 5 m grid still produced hundreds of points for bare-earth elevation on each terrace, thereby producing estimates for the topography that are comparable to calculations made using the full-resolution DEM.
From these elevations, the median value and interquartile range were found for each of the 52 mapped terraces. Since the Trinity River valley in the study area trends N–S, the elevation data for each terrace are plotted against median Universal Transverse Mercator (UTM) northing in Fig. 4a. The best-fit plane for the modern valley was used to generate detrended elevations for each terrace DEM measurement by subtracting it from the spatially corresponding modern valley best-fit plane value. This plane defining the modern valley floor was generated from a subsampled DEM with a 10 m grid resolution. The RMSE of the plane fit is 1.36 m, with most of the >4 500 000 points falling within 5 m of the plane. Plotting the residuals to the best-fit plane along UTM northing reveals some structure in the most downstream southern long profile extent (Fig. 4a insert), likely due to Holocene sedimentation (Blum et al., 1995; Blum and Aslan, 2006). However, we do not think this affects our detrended terrace analysis. The detrended median elevations and associated interquartile ranges for each terrace are presented in Fig. 4b. We then compared the distributions of detrended elevations for the terrace classifications. Each classified distribution, scaled to its contribution to the overall number of detrended elevations, is plotted in Fig. 5. Even though their median values are different, the detrended elevation distribution for the intermediate Deweyville terraces fully overlaps with that of the low Deweyville terraces (Fig. 5).
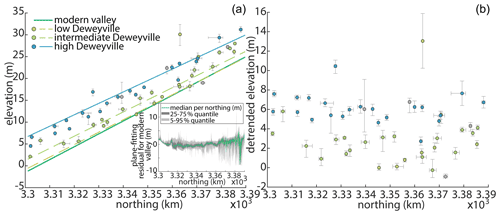
Figure 4(a) Median terrace elevation and (b) detrended elevation for the 52 terraces along the N–S-trending valley, colored by terrace category according to Blum (1995) and Garvin (2008) as high, intermediate, and low Deweyville. Terraces not previously identified were left grey. The error bars represent the interquartile range around the median terrace UTM and elevation values. The dark green line corresponds to the plane fitted to the 10 m DEM of modern valley elevations and the insert shows the residual of this plane fit. Blue, green, and light green lines indicate the plane fit to 1 m DEM terrace elevations assigned to each Deweyville terrace category.
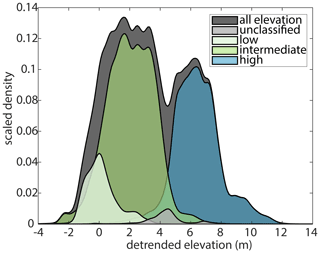
Figure 5Distributions of detrended elevations for terraces classified by Garvin (2008). Distributions were generated using a Gaussian kernel with a bandwidth of 0.2 and scaled by the proportion of the total elevation points (164 520) present in each classification. There are 16 543, 84 784, 60 244, and 2960 points in the low, intermediate, high, and unclassified groupings, respectively. There is complete overlap between the detrended elevations of low and intermediate terraces. Terraces classified as high and intermediate have less overlap. The median detrended elevations for the low, intermediate, high, and unclassified Deweyville groupings are 0.3, 2.05, 6.3, and 4.41 m, respectively.
4.1 Testing terrace formation using elevation data
We began our hypothesis testing by determining the best-fit plane to all of the raw elevation points (x, y, z) for terraces classified into the three Deweyville groups by Blum et al. (1995) and Garvin (2008) using a linear least-squares method. A planar surface was chosen for this analysis because the modern river surface and valley profiles are near linear in our area of study (Fig. 4a). The goodness of fit for these three planes to their associated terrace data was captured by the root-mean-square error (RMSE), which provides a measure of average variability of actual terrace elevations about the best-fit plane (Fig. 6). The next step was to compare the properties of these fitted planes against planes fit to terraces randomly drawn from the overall population. The randomly assigned terraces were put into one of three groups that had the same number of elements as the classified groups of high (n=22), middle (n=19), and low (n=8) Deweyville terraces. Best-fit planes were calculated and their RMSE was recorded. This process of randomly assigning terraces into three groups was then repeated 50 000 times to derive a large dataset of elevations, characterizing randomly grouped terraces (Fig. 7).
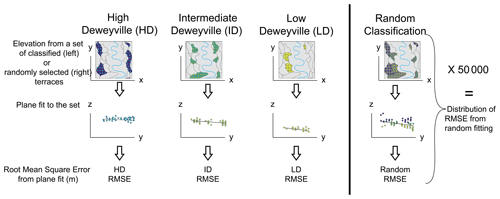
Figure 6Method to determine if classifications assigned to terraces represent distinct terrace groups. A plane was first fit to elevations extracted from the classified terrace groups in Garvin (2008) at a 5 m grid resolution (shown to the left of the vertical black line). We then fit planes to three randomly grouped sets of terraces using the same elevation data, iterating 50 000 times, for a total of 150 000 fits (shown to the right of the vertical black line). The root-mean-square error (RMSE) of the plane fit from each of the previously classified terrace groups was compared to the distribution of RMSE of the randomly grouped terraces (Fig. 7).
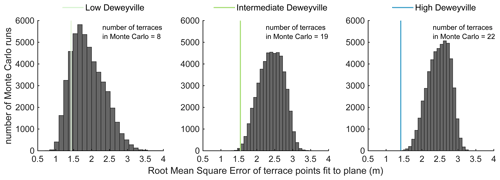
Figure 7Root-mean-square error (RMSE) of a plane fit to elevation points of terraces previously classified as high Deweyville, intermediate Deweyville, and low Deweyville in the Trinity River valley compared to a distribution of RMSE from 150 000 randomly grouped terraces. The low, intermediate, and high Deweyville terrace sets have RMSEs of 1.43, 1.54, and 1.41 m, respectively. All of the Deweyville classifications (light green, green, and blue lines) have an RMSE that falls within the distribution of RMSE for randomly grouped terraces. The high Deweyville classification is the closest to falling outside of the distribution, ∼ 3.4 standard deviations away from the random terraces RMSE distribution mean of 2.47 m (22 terrace groupings). The low and intermediate Deweyville classifications are ∼ 1.0 and ∼ 2.5 standard deviations away from the RMSE distribution mean of 1.89 and 2.40 m for 8 and 19 terrace groupings, respectively.
4.2 Evaluating terrace formation using paleo-channel analysis
Change in the discharge of the Trinity River during the late Pleistocene was estimated using the 79 mapped segments of paleo-channels preserved on terrace surfaces. Mean bankfull width (Bbf) for each paleo-channel mapped on the bare-earth DEM (Fig. 1b) was calculated from measurements extracted at 10 m intervals along each paleo-channel centerline (Fig. 8). Representative sidewall slopes (rise/run) for these paleo-channels range between 0.02 and 0.26 (Fig. 8a). These paleo-sidewall slopes fall within the range of modern sidewall slopes measured for the Trinity River in the study area by Smith and Mohrig (2017, their Fig. 5). Therefore, we confidently use the paleo-channel widths extracted from the DEM without any correction to the widths associated with relaxation of the paleo-topography over time. These data were used to estimate a formative, bankfull discharge (Qbf) for sand-bed rivers following the hydraulic geometry relationship developed by Wilkerson and Parker (2011):
where ν is the kinematic viscosity of water, R is the specific gravity of the sediment (), ρs is sediment density, ρ is water density, g is gravitational acceleration, and D50 is the median grain size of transported bed material. We used a value of 2650 kg m−3 for ρs and a range of paleo-channel grain sizes taken from Garvin (2008), who sampled both the lower and upper portions of bar deposits within preserved channel fills (Table 1). The uncertainty in estimated discharge was quantified for each paleo-channel using Monte Carlo simulation. For each run of the simulation, we sampled from (1) normal distributions with the reported means and standard deviations for each exponent in Eq. (1), (2) a normal distribution for channel width using its measured mean and standard deviation, and (3) a uniform distribution of grain sizes constrained by measurements from each classified terrace set (Table 1). This Monte Carlo simulation was run 50 000 times for each paleo-channel. Paleo-discharge estimates derived for the 79 channel segments preserved on terrace surfaces are plotted as a function of median detrended terrace elevation in Fig. 9.
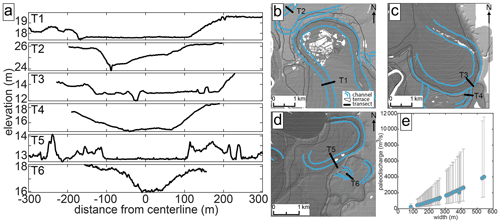
Figure 8Paleo-channel widths and paleo-discharge estimates. (a) Elevation transects for six paleo-channels (T1–T6). Transects are taken from locations indicated in (b)–(d) with mapped paleo-channels outlined in blue and mapped terrace extents outlined in grey. (e) Paleo-discharge estimates for the Trinity River are plotted as a function of their width. Each paleo-discharge was calculated using preserved channel width measurements and the discharge–width relationship from Wilkerson and Parker (2011) (Eq. 1). Error bars represent the first and third quartile of paleo-channel discharge estimates.
Table 1Grain size of terrace deposits from Garvin (2008) used for discharge calculations. The average grain size was calculated using the phi (logarithmic) scale.

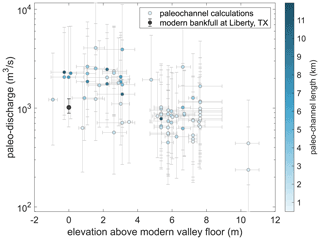
Figure 9Paleo-discharge estimates for the Trinity River plotted as a function of their associated detrended terrace elevations. Detrended elevations afford a crude stratigraphy for the discharges with the highest relative elevations representing older channels and the lowest elevations representing younger channels. Each paleo-discharge was calculated using preserved channel width measurements and the discharge–width relationship from Wilkerson and Parker (2011) (Eq. 1). Error bars represent the first and third quartile of paleo-channel discharge estimates and terrace elevations above the modern valley. The symbol was shaded to the preserved length for each paleo-channel, with darker symbols equated to longer segments. The modern bankfull discharge at Liberty, TX, was found using the methods described in the text and plotted at 0 m.
The accuracy of the Wilkerson and Parker (2011) relationship for the Trinity River system was tested by calculating a Qbf value for the modern river channel and comparing it against the bankfull discharge logged at the USGS gauge 08067000 at Liberty, Texas. The calculated bankfull discharge was estimated using the measured bankfull width of 170 m from the DEM at the gauge site. The median particle size of bed material at Liberty has been measured at 200 µm by the Trinity River Authority of Texas (Trinity River Authority of Texas, 2017). All other variables in Eq. (1) were kept constant between the modern river and paleo-channels, yielding an estimate for the modern bankfull discharge of 830 m3 s−1. The reported residual standard error associated with the bankfull discharge Eq. (1) (Wilkerson and Parker, 2011) was then used to approximate the error associated with this modern calculated bankfull discharge. The lower and upper standard error define a possible range between 340 and 2030 m3 s−1. These discharges estimated with Eq. (1) compare favorably with the measured discharge found using the rating curve for the USGS Liberty gauge station (https://waterdata.usgs.gov/nwisweb/data/ratings/exsa/USGS.08067000.exsa.rdb, last access: 20 June 2022) and bank-line elevations for the swath of channel extending 300 m both upstream and downstream of the gauge. The mean and standard deviation of bank elevations for this swath was 7.68 and 0.34 m, yielding a mean bankfull discharge of 1017 m3 s−1 and discharges of 887 and 1243 m3 s−1 corresponding to stages ±1 standard deviation in bank elevation.
4.3 Mixing models and bend cutoff analyses
To test the existence of statistical groupings within our terrace and paleo-channel data, a mixing model was used to generate Gaussian mixture distributions that were fitted to both the 164 520 detrended terrace elevation points and the median discharges estimated for the 79 paleo-channel segments (Fig. 10). Results are used to determine if Deweyville terraces should be divided into three distinct sets. The Akaike information criterion (AIC) and Bayesian information criterion (BIC) were applied to both mixing models in order to optimize the number of components used to represent each distribution (Fig. 10c and d). Two and three components were selected for the distributions of detrended elevation points and median paleo-channel discharges, respectively (Fig. 10a and b).
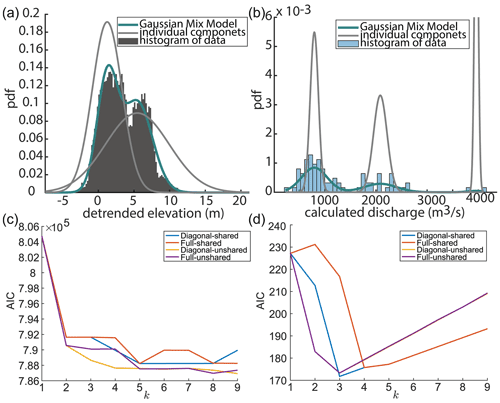
Figure 10Mixing model fits to measured distributions of terrace elevation and estimated paleo-discharges. Distributions using (a) elevation and (b) paleo-channels support an interpretation of allogenic forcing for high terrace abandonment due to increasing discharge. Akaike information criterion (AIC) for (c) detrended elevation and (d) paleo-discharge mixing model. BIC results are not shown here but have similar trends to AIC. AIC results are shown for the mixing model that are solved for a diagonal and full covariance matrix and shared and unshared covariance. The model also used a small regularization value to ensure the estimated covariance matrix is positive.
An important additional measurement used to assess whether terraces were abandoned due to enhanced local incision driven by gradient change during channel bend cutoff was the elevation differences between 40 adjacent terraces. These connections can first be assessed by comparing the minimum bounding box length of terraces, paleo-channel width, and paleo-channel length (Fig. 11). These measured elevation differences between terraces were compared to estimated elevation changes produced by bend cutoffs. We used Eq. (2) to calculate the elevation drop produced by a bend cutoff as follows:
On several low, intermediate, and high Deweyville terraces, the lengths of paleo-channels that had one bend preserved were measured using the bare-earth DEM (e.g., Fig. 2). The mean and standard deviations for bend lengths on the low, intermediate, and high terraces are 5.7 ± 2.8 km (n=3), 4.6 ± 3.0 km (n=10), and 2.3 ± 1.1 km (n=11), respectively. The overall distribution above the modern valley floor of paleo-channel lengths plotted in Fig. 11b. We approximated channel slope using the planes fit to the terrace elevation points for each classification. The calculated mean slope and standard error for the low, intermediate, and high terraces are 3.0 × 10−4 (3.1 × 10−6), 2.9 × 10−4 (1.10 × 10−6), and 3.0 × 10−4 (1.2 × 10−6), respectively. Using Eq. (2), estimated elevation drops driven by a possible bend cutoff are 1.6 ± 0.8, 1.3 ± 0.9, and 0.7 × 0.3 m (Fig. 12a).
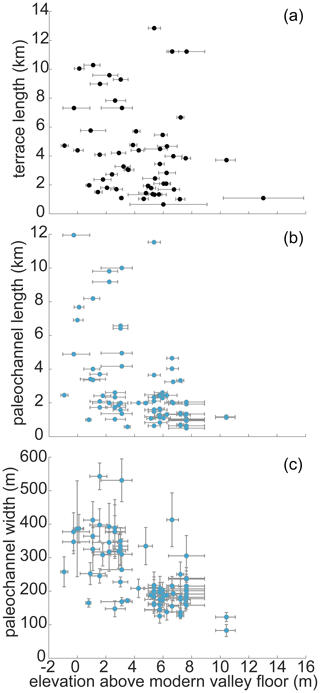
Figure 11(a) Terrace length, (b) paleo-channel length, and (c) paleo-channel width plotted along the median elevation of the associated terrace above the modern valley floor plane. The youngest terraces are more likely to have larger terrace lengths as well as paleo-channel lengths. Terrace length was measured as the longest length of a minimum bounding box envelop for each terrace. These envelopes were defined with edges in the N–S and E–W direction. Error bars show the interquartile range of each terrace.
An additional measurement used to evaluate the likelihood of terraces being produced by bend cutoff was the largest number of channel bends present in a segment of paleo-channel preserved on a terrace surface. The number of channel bends preserved on terrace surfaces can be used as an indicator for autogenic versus allogenic processes, whereby allogenic terrace formation likely abandons larger paleo-floodplain sections, preserving multiple channel bends. For incising rivers, the autogenic cutoff of a single meander bend has been shown to be sufficient to produce the enhanced channel erosion required to elevate relatively small sections of the previous active floodplain above flood levels (Finnegan and Dietrich, 2011).
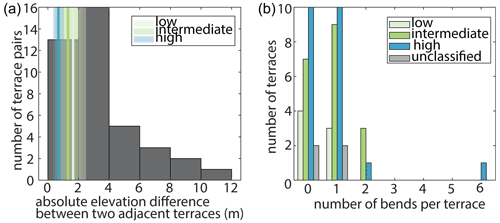
Figure 12Terrace properties used to assess the likelihood of meander bend cutoff being the driver of terrace formation. (a) Differences in elevation between adjacent terrace surfaces. Also plotted as vertical lines and swaths are the mean values ± 1 standard deviation for elevation decreases expected from cutting off a single meander bend for paleo-channels of the low, intermediate, and high Deweyville bounding surfaces. (b) Maximum number of paleo-meander bends preserved in a channel segment on each terrace. Most terraces have between 0–1 channel bends preserved for one generation of channel. Only intermediate and high Deweyville terraces have more than two channel bends preserved by a paleo-channel.
We mapped 52 terraces and 79 paleo-channel segments in the study area (Fig. 1b). Of these terraces, 22 are classified as high Deweyville, 19 as intermediate Deweyville, and 8 as low Deweyville, and 4 were left unclassified as they could not be correlated with terraces mapped by either Blum et al. (1995) or Garvin (2008). The low, intermediate, and high Deweyville terraces have median values for detrended elevations of 0.03, 2.06, and 6.37 m. Based on our mixture modeling, the mean and standard deviation of the detrended elevation components are 5.6 ± 4.18 and 1.32 ± 2.19 m with mixing proportions of 0.51 and 0.49, respectively. Similarly, the mean and standard deviation for the three Gaussian distributions describing paleo-discharges are 795 ± 80, 2083 ± 139, and 4013 ± 21 m3 s−1 with mixing proportions of 0.68, 0.30, and 0.02, respectively. Terraces vary in both size and shape, although they are typically elongate parallel to the valley axis and remain continuous for less than 10 km in that direction. The distribution of terraces is asymmetric, with more terraces observed on the east side of the valley (Fig. 1b). Consequently, most terraces are unpaired, meaning they have no topographic equivalent on the opposite side of the valley.
The best-fit planes to elevations for the Deweyville terrace groups defined by Blum et al. (1995), Garvin (2008), and Hidy et al. (2014) show remarkably similar slopes amongst terrace sets. The slopes and standard error for the low, intermediate, and high terraces are 3.0 × 10−4 (3.1 × 10−6), 2.9 × 10−4 (1.10 × 10−6), and 3.0 × 10−4 (1.2 × 10−6), respectively. These paleo-slopes are indistinguishable from the estimated slope for the modern valley of 3.0 × 10−4 (8.0 × 10−8) (Fig. 4a). It is not surprising that all four profiles are well fit by planes given the fact that the studied river segment represents less than 10 % of the modern river length and that both grain size and discharge vary little over the studied reach.
Plane fits for the low, intermediate, and high Deweyville terrace sets have RMSEs of 1.43, 1.54, and 1.41 m, respectively. Values of RMSE for best-fit planes to randomly grouped terraces are sensitive to the number of terraces defining a group. The fewer the number of terraces, the more likely it is that a low RMSE will result (Fig. 7). It is therefore important when comparing randomly grouped terraces to the previously classified groups that the number of terraces in each be the same. Running our analysis of 50 000 sets of randomly assembled terraces with the same number of elements as the low (n=8), intermediate (n=19), and high (n=22) Deweyville groups yielded the following RMSE results. The median and interquartile values of RMSE for planes fit to randomly selected terraces of the number present in the low-terrace classification are 1.82, 1.55, and 2.21 m. The median and interquartile values of RMSE for planes fit to randomly selected terraces of the number present in the intermediate-terrace classification are 2.41, 2.15, and 2.66 m. Finally, the median and interquartile values of RMSE for planes fit to randomly selected terraces of the number present in the high-terrace classification are 2.49, 2.25, and 2.71 m.
The RMSE values for the best-fit planes to the classified Deweyville terraces are plotted on their associated synthetic RMSE distributions for randomly selected terraces in Fig. 7. Inspection of Fig. 7 reveals little overlap between the classified high terraces and the random samplings of terraces. For the high Deweyville case, there was only a 0.008 % occurrence of randomly selected terraces yielding an RMSE as low as 1.41 m. A very different result was found for the classified low terraces, where its RMSE falls well within the associated distribution of synthetic RMSEs, with fully 21 % of all randomly selected cases having lower RMSE values. Minimal overlap was found between the RMSE for the classified intermediate terraces and the distribution of RMSE values generated from random terrace groupings. Only 1 % of the randomly selected sets of terraces were a better fit to a plane than the classified group of intermediate terraces. Mapped paleo-channels have widths that range from 82 to 543 m (Fig. 8). Estimated bankfull discharges calculated using these widths (Eq. 1) range from 233 m3 s−1 to more than 4000 m3 s−1 (Fig. 9). These paleo-discharges cluster into two groups, one at lower discharges centered around 795 ± 80 m3 s−1 and one at higher discharges centered on 2083 ± 139 m3 s−1 (Fig. 10b). The grouping of lower-discharge paleo-channels sit on terraces that have median elevations >4.5 m above the modern valley floor and correspond to high Deweyville terraces (Fig. 9). The grouping of higher-discharge paleo-channels is preserved on terraces that have median elevations from 0.2 m below to 5.2 m above the modern floodplain and correspond to both intermediate and low Deweyville terraces (Fig. 9). The investigation of paleo-channel characteristics revealed that paleo-channel widths, paleo-channel lengths, and overall terrace lengths all are more likely to be greater for younger terraces (Fig. 11). Most terraces have one or fewer channel bends preserved (Fig. 12b) and only the intermediate and high Deweyville classifications possess terraces with more than two preserved channel bends.
Late Pleistocene terraces of the lower Trinity River valley formed during a period of net sea-level fall punctuated by shorter and smaller magnitude fluctuations (Anderson et al., 2016). Previous researchers have interpreted the formation of the Trinity terraces, as well as those observed in other Texas coastal valleys, in the context of these fluctuations (Blum et al., 1995; Blum and Aslan, 2006; Morton et al., 1996; Rodriguez et al., 2005). However, it has also been suggested that this terrace formation in the lower Trinity River valley was driven by autogenic triggers (Guerit et al., 2020). The motivation for this study was to develop tools to help distinguish between these two forcings that can produce terraces.
Several morphological characteristics exist to describe both the Trinity River terraces and their associated paleo-channels. The terraces are most commonly unpaired (Fig. 1), which is expected during autogenic terrace formation associated with unsteady lateral migration rates during formation (Bull, 1990; Merritts et al., 1994) and river bend cutoff (Finnegan and Dietrich, 2011). On the flip side, paired terraces can also be formed during constant, albeit low vertical incision rates, during lateral migration (Limaye and Lamb, 2016). Unpaired terraces can also be produced by unequal lateral river erosion after the terrace formation that preferentially removes half of a previously formed pair of allogenic terraces (Malatesta et al., 2017). Similarly, lateral migration also affects the age distribution of the terraces preserved because younger terraces closer to the modern river are more likely to eroded away than older terraces (Lewin and Macklin, 2003; Limaye and Lamb, 2016). The presence of unpaired terraces in the lower Trinity River valley may therefore be most indicative of the relative importance of lateral migration for this system.
For the Trinity River, many of the valley-ward edges of the lower and intermediate Deweyville bounding surfaces have the shapes of meander bends, recording the most outward extent of the active channel before the floodplain surface was abandoned (Figs. 1, 2). We take this as evidence for the autogenic process of channel cutoff triggering terrace formation. The observed elevation differences between adjacent terraces are also consistent with those predicted by cutoff of a single meander bend (Fig. 12a). Similar interpretations have also been made for strath terraces in bedrock (Finnegan and Dietrich, 2011). Furthermore, their tendency to be preserved as unpaired terraces with a small number (<2) of channel bends is more consistent with the stochastic nature of meander cutoffs by autogenic processes than large-scale incisional events due to allogenic forcings (Fig. 12a and b, Finnegan and Dietrich, 2011). Therefore, the morphology of the Trinity River valley terraces alone is suggestive of an autogenic forcing, but it is likely not sufficient to distinguish between allogenic versus autogenic terrace formation.
We argue that a robust test for assessing the likelihood of autogenic versus allogenic forcing in terrace formation comes from an analysis of the topographic variability of terrace sets inferred to have formed synchronously. Here we have developed a method to quantitatively compare elevation variability of any classified group of terraces against randomly selected terrace sets (Figs. 6, 7) so that we can evaluate whether a classified group is better organized than arbitrarily selected ones. For the lower Trinity River valley, if the Deweyville terraces formed synchronously (Blum et al., 1995; Blum and Aslan, 2006; Morton et al., 1996; Rodriguez et al., 2005), one would predict that terraces within these groups would show lower variation about a best-fit plane than randomly grouped terraces (Fig. 7). Limaye and Lamb (2016) defined a unique elevation set as surfaces that are separated by more than 1 m. They found that lateral migration during a constant incision rate versus pulsed incision rates can result in similar and indistinguishable terrace sets (Limaye and Lamb, 2016). Our approach builds on this idea and develops a framework that evaluates the magnitudes of variations in elevation amongst terraces compared to a fitted plane for the set. This approach is especially useful for studies where age control across terraces is not well constrained. Since we are assessing many elevation points from each terrace in the terrace set, it is possible to tease apart long profile variations for terrace sets only vertically separated by ∼ 1 m.
Our RMSE results show that the best-fit plane for the low Deweyville bounding surface cannot be separated from, and is instead consistent with, sets of randomly grouped terraces mimicking autogenic processes of either bend cutoff or of unsteady river lateral migration during constant base level fall (Fig. 7b). The driver for the intermediate Deweyville bounding surface cannot be unambiguously determined based on the RMSE analysis. The classified group is better organized than most, but not all, randomly generated groupings of terraces (Fig. 7c). The overlap leads us to presume that the null hypothesis of autogenic terrace formation cannot be robustly falsified. A different conclusion was reached for the high Deweyville bounding surface. With our RMSE analysis, we reject the null hypothesis of autogenic terrace formation. The high Deweyville bounding surface is most likely the product of punctuated allogenic change with an RMSE that is as small as any of the 50 000 values generated for random groupings of terraces (Fig. 7a). A difference between the low or intermediate terraces and the high terraces was also found in the distribution of detrended terrace elevations using a two-component Gaussian mixing model. The first component of this model overlaps with elevations classified as low and intermediate Deweyville, while the second component corresponds most closely to high Deweyville elevations (Figs. 5, 10a). We therefore conclude that the high Deweyville terraces are different than the other two sets and record an allogenic signal connected with early valley incision. This new analysis likely means that across a relatively short interval of time, <10 kyr, terraces on the Trinity River switched from recording an allogenic trigger in the high Deweyville bounding surface to being indistinguishable from terraces formed by autogenic triggers such as bend cutoff or unsteady lateral migration rates.
The connections between potential discharge changes and terrace formation were assessed using paleo-channel widths and grain size (Figs. 9, 10b). Paleo-channel discharge estimates reveal a factor of 2 increase in bankfull discharge moving from older, high Deweyville terraces to younger, intermediate, and low Deweyville terraces. The estimated changes through time in bankfull discharge are not matched by estimated changes in river long-profile slopes or paleo-slopes. Previously discussed best-fit planes to the Deweyville bounding surface have slopes that are roughly constant and indistinguishable from the modern long profile for the Trinity River (Fig. 4a). Theory by Parker et al. (1998) and experiments by Whipple et al. (1998) have demonstrated that the long-profile slope for sandy fluvial systems is a function of sediment-to-water discharges. Terraces associated with base level fall have been shown to maintain consistent valley slopes (Tofelde et al., 2019). Experiments by the same authors also showed that sediment and/or water discharge changes produce changing slopes for terrace sets, which we do not observe here. We suspect that the switch in discharge is not directly recorded in the terrace elevation because the change in water discharge appears to have been approximately matched by a sediment flux increase, as recorded in the constant long-profile slope for the paleo-river. With no slope reduction, no incision would have occurred. As a result, discharge changes recorded by segments of paleo-channels on the intermediate and low Deweyville terraces are not interpreted to have driven incision and terrace formation. Instead, it likely that an autogenic trigger associated with persistent base level fall drove the terracing. Recent synthesis studies by Phillips and Jerolmack (2016) and Dunne and Jerolmack (2018) confirm that these estimates of bankfull discharge are tied to moderate flooding and representative of mean climate properties. While our estimated discharge changes over the latest Pleistocene are large, it is only half of the proposed 4 times increase reported for similar paleo-channels preserved on terraces of the nearby lower Brazos River valley (Sylvia and Galloway, 2006).
Maintenance of a roughly constant slope while water discharge changed therefore almost certainly required commensurate changes to sediment discharge. We can test this change in sediment discharge by looking at results from existing studies. An increase in sediment discharge is in agreement with Anderson (2005), who suggests that sediment discharge was greater during the LGM than today. However, calculations for the Trinity River by other authors do not currently reflect these changes. Sediment discharges have been estimated to decrease during the LGM (intermediate and low Deweyville) based on the BAQRT model by Syvitski and Milliman (2007) (Blum and Hattier-Womack, 2009; Garvin, 2008). Hidy et al. (2014) also calculated 10Be denudation rates and suggested that upstream weathering was greater during the interglacial periods and that reworking of stored sediments was greater during glacial periods. However, Hidy et al. (2014) was not able to combine the effects of reworking and upstream sediment flux using 10Be to estimate the sediment discharge associated with terrace formation. More recent methods were developed to estimate sediment discharge based on bedforms and stratigraphy, which are exposed along the Trinity River (Mahon and McElroy, 2018). Therefore, there is also an opportunity to refine and improve sediment discharge estimates for Deweyville terraces. Regardless, responsive adjustments to sediment discharge suggest that the river itself remained a predominantly transport-limited system throughout the latest Pleistocene (Howard, 1980; Whipple, 2002).
Understanding the cutoff of a river bend is important to identify autogenic triggers for terrace formation. We have shown that a majority of Deweyville terraces in the Trinity valley preserve no more than a single paleo-channel bend (Fig. 12b) and that elevation differences between adjacent terraces are similar to an expected elevation change driven by channel shortening through cutoff to a river bend (Fig. 12a). These terrace properties highlight an opportunity for our community to measure the number of bends involved in the autogenic shortening of river channels. Specifically, there is an opportunity to quantify what percentage of cutoffs result in two or more bends being detached from the active channel in short amounts of time, thereby refining an expected upper limit to the number of channel bends preserved on autogenically generated terraces. Exceptionally preserved paleo-channels, such as those on the Trinity River, provide this opportunity to distinguish autogenic processes responsible for terrace formation, and as such might provide a more faithful record of changes in discharge to the system than terrace elevations and morphologies. An additional mechanism for reducing uncertainty in the processes that cut Trinity terraces would be assembling a greater number of terrace ages. Increasing age control could constrain vertical versus lateral migration rates for the river to a point where autogenic versus allogenic processes connected to terrace formation are separable (Limaye and Lamb, 2016; Merritts et al., 1994).
Irrespective of terrace formation, other river systems across the southeastern United States have the potential to also record a step increase in formative discharge seen in the Trinity River valley between the high to intermediate (or low) Deweyville terraces. This change was likely driven by a wetter climate in southeastern Texas during the period ∼ 34–20 ka based on OSL dates for the low and intermediate Deweyville terraces (Garvin, 2008). During the Last Glacial Maximum (19–26 ka), precipitation in western and southwestern USA has been shown to be ∼ 0.75–1.5 and ∼ 1.3–1.6 of modern values, respectively (Ibarra et al., 2018). Additionally, general circulation model (GCM) models show a general increase in precipitation in the study area during the late Pleistocene (Roberts et al., 2014 (Fig. 2); McGee et al., 2018 (Fig. 2)). Our observations agree with other workers who interpreted the changes in channel size as an increase in mean discharge during this period (Alford and Holmes, 1985; Gagliano and Thom, 1967; Saucier and Fleetwood, 1970; Sylvia and Galloway, 2006). Observations of larger paleo-channels during this period are also seen across rivers in the southeast of Texas (e.g., Bernard, 1950; Blum et al., 1995; Sylvia and Galloway, 2006), Arkansas and Louisiana (e.g., Saucier and Fleetwood, 1970), and Georgia and South Carolina (e.g., Leigh and Feeney, 1995; Leigh et al., 2004; Leigh, 2008).
Our contribution to the existing work on terraces in this region is to reconcile the literature that suggests an episodic cut and fill and/or base level change model (Blum et al., 1995) with the literature on terrace formation due to increased discharge (Sylvia and Galloway, 2006). While both have the potential to generate terraces, intrinsic processes such as bend cutoff and unsteady lateral migration during constant base level fall need to first be ruled out. For example, relatively slow vertical incision rates, pulsed discharge changes (allogenic process), and unsteady lateral migration (autogenic process) showed indistinguishable morphologies in Limaye and Lamb (2016). Here, for all but the high Deweyville, autogenic triggers for terrace development cannot be ruled out.
The results presented here demonstrate that it is critical to understand the many potential forcings (both allogenic and autogenic) on a river system that can lead to terrace formation and to employ robust, quantitative tests for discriminating between these forcings before using terraces to reconstruct paleo-environmental histories. The method proposed here for assessing the role of allogenic processes in terrace formation using the variability of terrace elevations provides a simple, quantitative test and may prove useful for interpreting terrace formation in other river systems. We were not able to correlate terrace levels back to distinct trigger events, although allogenic forcings such as sea level fluctuations and discharge changes were also classified here. We suggest that paleo-channel characteristics are a more faithful record of discharge changes in fluvial systems and that additional bend metrics can differentiate autogenic terrace formation processes, specifically bend cutoff from unsteady lateral migration rates.
The code to reproduce figures can be found at https://doi.org/10.5281/zenodo.6620600 (Hassenruck-Gudipati, 2022).
The lidar dataset was acquired from the Texas Natural Resource Information System (TNRIS) at https://tnris.org (Strategic Mapping Program, 2017a, b, 2018; FEMA, 2011). Please see the reference to each dataset. Tables with analysis produced from the lidar datasets are included in the Supplement.
The supplement related to this article is available online at: https://doi.org/10.5194/esurf-10-635-2022-supplement.
All authors designed the analysis and contributed to the manuscript writing. HJHG and TE analyzed the lidar dataset. TAG developed the code to fit planes to the lidar dataset.
The contact author has declared that neither they nor their co-authors have any competing interests.
Publisher's note: Copernicus Publications remains neutral with regard to jurisdictional claims in published maps and institutional affiliations.
We thank Webster Mangham for providing the report from the Trinity River Authority of Texas Phase II, Bathymetry and Sediment Collection for the Port of Liberty Study. We thank Ajay Limaye and one anonymous reviewer for their constructive reviews. We also thank Joel Johnson and Gary Kocurek for insightful comments on early versions of the manuscript and Niels Hovius, Mike Lamb, Paola Passalacqua, and Daniella Rempe for additional comments.
This research has been supported by the U.S. National Science Foundation Graduate Research Fellowship Program (grant no. DGE-1610403) and the Jackson School of Geosciences, The University of Texas at Austin.
This paper was edited by Sebastien Castelltort and reviewed by Ajay Limaye and one anonymous referee.
Alford, J. J. and Holmes, J. C.: Meander Scars as Evidence of Major Climate Change in Southwest Louisiana, Ann. Assoc. Am. Geogr., 75, 395–403, https://doi.org/10.1111/j.1467-8306.1985.tb00074.x, 1985.
Anderson, J. B.: Diachronous development of Late Quaternary shelf-margin deltas in the northwestern Gulf of Mexico: implications for sequence stratigraphy and deep water reservoir occurrence, in: River Deltas: Concepts, Models and Examples, edited by: Giosan, L. and Bhattacharya, J. P., SEPM Society for Sedimentary Geology, Vol. 83, 257–276, https://doi.org/10.2110/pec.05.83, 2005.
Anderson, J. B., Wallace, D. J., Simms, A. R., Rodriguez, A. B., and Milliken, K. T.: Variable response of coastal environments of the northwestern Gulf of Mexico to sea-level rise and climate change: Implications for future change, Mar. Geol., 352, 348–366, https://doi.org/10.1016/j.margeo.2013.12.008, 2014.
Anderson, J. B., Wallace, D. J., Simms, A. R., Rodriguez, A. B., Weight, R. W. R., and Taha, Z. P.: Recycling sediments between source and sink during a eustatic cycle: Systems of late Quaternary northwestern Gulf of Mexico Basin, Earth-Sci. Rev.., 153, 111–138, https://doi.org/10.1016/j.earscirev.2015.10.014, 2016.
Baker, E. T. J.: Stratigraphic Nomenclature and Geologic Sections of the Gulf, U.S. Geol. Surv. Open-File Rep., 34, 94–461, 1995.
Bernard, H. A.: Quaternary Geology of Southeast Texas, Louisiana State University, Historical Dissertations and Theses 7952, 1950.
Blom, A., Arkesteijn, L., Chavarrías, V., and Viparelli, E.: The equilibrium alluvial river under variable flow and its channel-forming discharge, J. Geophys. Res.-Ea. Surf., 122, 1924–1948, https://doi.org/10.1002/2017JF004213, 2017.
Blum, M., Martin, J., Milliken, K., and Garvin, M.: Paleovalley systems: Insights from Quaternary analogs and experiments, Earth-Sci. Rev., 116, 128–169, https://doi.org/10.1016/j.earscirev.2012.09.003, 2013.
Blum, M. D. and Aslan, A.: Signatures of climate vs. sea-level change within incised valley-fill successions: Quaternary examples from the Texas GULF Coast, Sediment. Geol., 190, 177–211, https://doi.org/10.1016/j.sedgeo.2006.05.024, 2006.
Blum, M. D. and Hattier-Womack, J., Climate Change, Sea-Level Change, and Fluvial Sediment Supply to Deepwater Depositional Systems, External Controls of Deep-Water Depositional Systems, 15–39, https://doi.org/10.2110/SEPMSP.092.015, 2009.
Blum, M. D. and Törnqvist, T. E.: Fluvial responses to climate and sea-level change: A review and look forward, Sedimentology, 47, 2–48, https://doi.org/10.1046/j.1365-3091.2000.00008.x, 2000.
Blum, M. D., Morton, R. A., and Durbin, J. M.: “Deweyville” Terraces and Deposits of the Texas Gulf Coastal Plain, GCAGS Trans., 45, 53–60, 1995.
Bull, W. B.: Stream-terrace genesis: implications for soil development, Geomorphology, 3, 351–367, https://doi.org/10.1016/0169-555X(90)90011-E, 1990.
Church, M.: Bed Material Transport and the Morphology of Alluvial River Channels, Annu. Rev. Earth Planet. Sci., 34, 325–354, https://doi.org/10.1146/annurev.earth.33.092203.122721, 2006.
Daley, J. and Cohen, T.: Climatically-Controlled River Terraces in Eastern Australia, Quaternary, 1, 23–43, https://doi.org/10.3390/quat1030023, 2018.
Dunne, K. B. J. and Jerolmack, D. J.: Evidence of, and a proposed explanation for, bimodal transport states in alluvial rivers, Earth Surf. Dynam., 6, 583–594, https://doi.org/10.5194/esurf-6-583-2018, 2018.
Erkens, G., Dambeck, R., Volleberg, K. P., Bouman, M. T. I. J., Bos, J. A. A., Cohen, K. M., Wallinga, J., and Hoek, W. Z.: Fluvial terrace formation in the northern Upper Rhine Graben during the last 20 000 years as a result of allogenic controls and autogenic evolution, Geomorphology, 103, 476–495, https://doi.org/10.1016/j.geomorph.2008.07.021, 2009.
FEMA: FEMA 2011 1m Liberty Lidar, TNRIS [data set], https://data.tnris.org/collection?c=2407d7a6-c33a-4095-915d-ac464293ef50#8.85/30.188/-94.875, last access: 20 June 2022, 2011.
Finnegan, N. J. and Dietrich, W. E.: Episodic bedrock strath terrace formation due to meander migration and cutoff, Geology, 39, 143–146, https://doi.org/10.1130/G31716.1, 2011.
Gagliano, S. M. and Thom, B. G.: Deweyville Terrace, Gulf and Atlantic Coasts Technical Report 39, Baton Rouge, 1967.
Galloway, W. E., Ganey-Curry, P. E., Li, X., and Buffler, R. T.: Cenozoic depositional history of the Gulf of Mexico basin, Am. Assoc. Pet. Geol. Bull., 84, 1743–1774, https://doi.org/10.1306/8626C37F-173B-11D7-8645000102C1865D, 2000.
Garvin, M. G.: Late Quaternary geochronologic, stratigraphic, and sedimentologic framework of the Trinity River incised valley: East Texas coast, December, LSU Master's Theses, 1122, https://doi.org/10.31390/gradschool_theses.1122, 2008.
Guerit, L., Foreman, B. Z., Chen, C., Paola, C., and Castelltort, S.: Autogenic delta progradation during sea-level rise within incised valleys, Geology, 49, 1–5, https://doi.org/10.1130/g47976.1, 2020.
Hancock, G. S. and Anderson, R. S.: Numerical modelling of fluvial strath terrace formation in response to oscillating climate, Geol. Soc. Am. Bull., 114, 1131–1142, https://doi.org/10.1130/0016-7606(2002)114<1131, 2002.
Hassenruck-Gudipati, H. J.: Trinity Terraces: manuscript figures, Zenodor [code], https://doi.org/10.5281/zenodo.6620600, 2022.
Heinrich, P., Miner, M., Paulsell, R., and McCulloh, R.: Response of Late Quaternary Valley systems to Holocene sea level rise on continental shelf offshore Louisiana: preservation potential of paleolandscapes, New Orleans (LA), US Department of the Interior, Bureau of Ocean Energy Management, Report No.: BOEM 2020-004, 2020.
Hidy, A. J., Gosse, J. C., Blum, M. D., and Gibling, M. R.: Glacial-interglacial variation in denudation rates from interior Texas, USA, established with cosmogenic nuclides, Earth Planet. Sci. Lett., 390, 209–221, https://doi.org/10.1016/j.epsl.2014.01.011, 2014.
Howard, A. D.: Thresholds in river regimes, in: Thresholds in geomorphology, edited by: Coates, D. R. and Vitek, J. D., Binghamton Geomorphology Symposium 9, 1st Edn., Routledge, 227–258, https://doi.org/10.4324/9781003028697, 1980.
Ibarra, D. E., Oster, J. L., Winnick, M. J., Rugenstein, J. K. C., Byrne, M. P., and Chamberlain, C. P.: Warm and cold wet states in the western United States during the Pliocene-Pleistocene, Geology, 46, 355–358, https://doi.org/10.1130/G39962.1, 2018.
Knox, J. C.: Responses of floods to Holocene climatic change in the upper Mississippi Valley, Quat. Res., 23, 287–300, https://doi.org/10.1016/0033-5894(85)90036-5, 1985.
Lane, E. W.: Design of Stable Channels, Trans. Am. Soc. Civ. Eng., 120, 1234–1260, https://doi.org/10.1061/TACEAT.0007188, 1955.
Leigh, D. S.: Late Quaternary climates and river channels of the Atlantic Coastal Plain, Southeastern USA, Geomorphology, 101, 90–108, https://doi.org/10.1016/j.geomorph.2008.05.024, 2008.
Leigh, D. S. and Feeney, T. P.: Paleochannels indicating wet climate and lack of response to lower sea level, southeast Georgia, Geology, 23, 687–690, https://doi.org/10.1130/0091-7613(1995)023< 0687:PIWCAL>2.3.CO;2, 1995.
Leigh, D. S., Srivastava, P., and Brook, G. A.: Late Pleistocene braided rivers of the Atlantic Coastal Plain, USA, Quat. Sci. Rev., 23, 65–84, https://doi.org/10.1016/S0277-3791(03)00221-X, 2004.
Lewin, J. and Macklin, M. G.: Preservation potential for late quaternary river alluvium, J. Quat. Sci., 18, 107–120, https://doi.org/10.1002/jqs.738, 2003.
Limaye, A. B. S. and Lamb, M. P.: Numerical simulations of bedrock valley evolution by meandering rivers with variable bank material, J. Geophys. Res.-Ea. Surf., 119, 927–950, https://doi.org/10.1002/2013JF002997, 2014.
Limaye, A. B. S. and Lamb, M. P.: Numerical model predictions of autogenic fluvial terraces and comparison to climate change expectations, J. Geophys. Res.-Ea. Surf., 121, 512–544, https://doi.org/10.1002/2014JF003392, 2016.
Mackey, B. H., Roering, J. J., and Lamb, M. P.: Landslide-dammed paleolake perturbs marine sedimentation and drives genetic change in anadromous fish, P. Natl. Acad. Sci. USA, 108, 18905–18909, https://doi.org/10.1073/pnas.1110445108, 2011.
Mahon, R. C. and McElroy, B.: Indirect estimation of bedload flux from modern sand-bed rivers and ancient fluvial strata, Geology, 46, 579–582, https://doi.org/10.1130/G40161.1, 2018.
Malatesta, L. C., Prancevic, J. P., and Avouac, J. P.: Autogenic entrenchment patterns and terraces due to coupling with lateral erosion in incising alluvial channels, J. Geophys. Res.-Ea. Surf., 122, 335–355, https://doi.org/10.1002/2015JF003797, 2017.
McGee, D., Moreno-Chamarro, E., Marshall, J., and Galbraith, E. D.: Western U.S. lake expansions during Heinrich stadials linked to Pacific Hadley circulation, Sci. Adv., 4, 1–11, https://doi.org/10.1126/sciadv.aav0118, 2018.
Merritts, D. J., Vincent, K. R., and Wohl, E. E.: Long river profiles, tectonism, and eustasy: A guide to interpreting fluvial terraces, J. Geophys. Res.-Solid Earth, 99, 14031–14050, https://doi.org/10.1029/94JB00857, 1994.
Molnar, P., Brown, E. T., Burchfiel, B. C., Deng, Q., Feng, X., Li, J., Raisbeck, G. M., Shi, J., Zhangming, W., Yiou, F., and You, H.: Quaternary Climate Change and the Formation of River Terraces across Growing Anticlines on the North Flank of the Tien Shan, China, J. Geol., 102, 583–602, https://doi.org/10.1086/629700, 1994.
Morton, R. A., Blum, M. D., and White, W. A.: Valley Fills of Incised Coastal Plain Rivers, Southeastern Texas, Gulf Coast Assoc. Geol. Soc., 46, 321–331, https://doi.org/10.1306/2DC40B2D-0E47-11D7-8643000102C1865D, 1996.
Muto, T. and Steel, R. J.: Autogenic response of fluvial deltas to steady sea-level fall: Implications from flume-tank experiments, Geology, 32, 401–404, https://doi.org/10.1130/G20269.1, 2004.
Parker, G., Paola, C., Whipple, K. X., and Mohrig, D.: Alluvial Fans Formed by Channelized Fluvial and Sheet Flow. I: Theory, J. Hydraul. Eng., 124, 985–995, https://doi.org/10.1061/(ASCE)0733-9429(1998)124:10(985), 1998.
Pazzaglia, F. J.: Fluvial Terraces, in: Treatise on Geomorphology, vol. 9, edited by: Shroder, J. and Wohl, E., Academic Press, 379–412, https://doi.org/10.1016/B978-0-12-374739-6.00248-7, 2013.
Pazzaglia, F. J. and Gardner, T. W.: Fluvial terraces of the lower Susquehanna River, Geomorphology, 8, 83–113, https://doi.org/10.1016/0169-555X(93)90031-V, 1993.
Pazzaglia, F. J., Gardner, T. W., and Merritts, D. J.: Bedrock fluvial incision and longitudinal profile development over geologic time scales determined by fluvial terraces, in: Rivers Over Rock: Fluvial Processes in Bedrock Channels, edited by: Tinkler, K. J. and Wohl, E. E., 207–235, https://doi.org/10.1029/GM107p0207, 1998.
Phillips, C. B. and Jerolmack, D. J.: Self-organization of river channels as a critical filter on climate signals, Science, 352, 694–697, https://doi.org/10.1126/science.aad3348, 2016.
Poisson, B. and Avouac, J. P.: Holocene hydrological changes inferred from alluvial stream entrenchment in North Tian Shan (Northwestern China), J. Geol., 112, 231–249, https://doi.org/10.1086/381659, 2004.
Roberts, W. H. G., Valdes, P. J., and Payne, A. J.: Topography's crucial role in Heinrich Events, P. Natl. Acad. Sci. USA, 111, 16688–16693, https://doi.org/10.1073/pnas.1414882111, 2014.
Rodriguez, A. B., Anderson, J. B., and Simms, A. R.: Terrace Inundation as an Autocyclic Mechanism for Parasequence Formation: Galveston Estuary, Texas, U.S.A., J. Sediment. Res., 75, 608–620, https://doi.org/10.2110/jsr.2005.050, 2005.
Saucier, R. T.: Geomorphology and Quaternary Geologic History of the Lower Mississippi Valley, US Army Corps Eng., I, 1–414, 1994.
Saucier, R. T. and Fleetwood, A. R.: Origin and Chronologic Significance of Late Quaternary Terraces, Ouachita River, Arkansas and Louisiana, GSA Bull., 81, 869–890, https://doi.org/10.1130/0016-7606(1970)81[869:OACSOL]2.0.CO;2, 1970.
Simms, A. R., Anderson, J. B., Milliken, K. T., Taha, Z. P., and Wellner, J. S.: Geomorphology and age of the oxygen isotope stage 2 (last lowstand) sequence boundary on the northwestern Gulf of Mexico continental shelf, Geol. Soc. Spec. Publ., 277, 29–46, https://doi.org/10.1144/GSL.SP.2007.277.01.03, 2007.
Smith, V. B. and Mohrig, D.: Geomorphic signature of a dammed Sandy River: The lower Trinity River downstream of Livingston Dam in Texas, USA, Geomorphology, 297, 122–136, https://doi.org/10.1016/j.geomorph.2017.09.015, 2017.
Spratt, R. M. and Lisiecki, L. E.: A Late Pleistocene sea level stack, Clim. Past, 12, 1079–1092, https://doi.org/10.5194/cp-12-1079-2016, 2016.
Strategic Mapping Program (StratMap): Jefferson, Liberty, and Chambers Counties Lidar, TNRIS [data set], https://data.tnris.org/collection/12342f12-2d74-44c4-9f00-a5c12ac2659c, last access: 20 June 2022, 2017a.
Strategic Mapping Program (StratMap): East Texas Lidar, TNRIS [data set], https://data.tnris.org/collection/f09f36b9-12b1-4c88-bccb-017afa9bc3d8, last access: 20 June 2022, 2017b.
Strategic Mapping Program (StratMap): Upper Coast Lidar, TNRIS [data set], https://data.tnris.org/collection/b5bd2b96-8ba5-4dc6-ba88-d88133eb6643, last access: 20 June 2022, 2018c.
Strong, N. and Paola, C.: Fluvial Landscapes and Stratigraphy in a Flume, Sediment. Rec., 4, 4–8, https://doi.org/10.2110/sedred.2006.2.4, 2006.
Syvitski, J. P. M. and Milliman, J. D.: Geology, Geography, and Humans Battle for Dominance over the Delivery of Fluvial Sediment to the Coastal Ocean, J. Geol., 115, 1–19,https://doi.org/10.1086/509246, 2007.
Sylvia, D. A. and Galloway, W. E.: Morphology and stratigraphy of the late Quaternary lower Brazos valley: Implications for paleo-climate, discharge and sediment delivery, Sediment. Geol., 190, 159–175, https://doi.org/10.1016/j.sedgeo.2006.05.023, 2006.
Thomas, M. A. and Anderson, J. B.: Sea-Level Controls on the Facies Architecture of the Trinity/Sabine Incised-Valley System, Texas Continental Shelf, in Incised-Valley Systems: Origin and Sedimentary Sequences, SEPM Society for Sedimentary Geology, edited by: Dalrymple, R. W., Ron Boyd, R., and Zaitlin, B. A., https://doi.org/10.2110/pec.94.12.0063, 1994.
Tofelde, S., Savi, S., Wickert, A. D., Bufe, A., and Schildgen, T. F.: Alluvial channel response to environmental perturbations: fill-terrace formation and sediment-signal disruption, Earth Surf. Dynam., 7, 609–631, https://doi.org/10.5194/esurf-7-609-2019, 2019.
Trinity River Authority of Texas: Phase II, Bathymetry and Sediment Collection for Port of Liberty Study, Webster Mangham, Trinity River Authority of Texas [data set], 2017.
U.S. Geological Survey: National Water Information System data available on the World Wide Web (USGS Water Data for the Nation), https://waterdata.usgs.gov/usa/nwis/uv?08066500 (last access: 20 June 2022), 2020a.
U.S. Geological Survey: National Water Information System data available on the World Wide Web (USGS Water Data for the Nation), https://waterdata.usgs.gov/nwis/uv?site_no=08067000 (last access: 20 June 2022), 2020b.
Wegmann, K. W. and Pazzaglia, F. J.: Holocene strath terraces, climate change, and active tectonics: The Clearwater River basin, Olympic Peninsula, Washington State, Bull. Geol. Soc. Am., 114, 731–744, https://doi.org/10.1130/0016-7606(2002)114<0731:HSTCCA>2.0.CO;2, 2002.
Whipple, K. X.: Implications of sediment-flux-dependent river incision models for landscape evolution, J. Geophys. Res., 107, 2039, https://doi.org/10.1029/2000JB000044, 2002.
Whipple, K. X., Parker, G., Paola, C., and Mohrig, D.: Channel Dynamics, Sediment Transport, and the Slope of Alluvial Fans: Experimental Study, J. Geol., 106, 677–694, https://doi.org/10.1086/516053, 1998.
Wickert, A. D. and Schildgen, T. F.: Long-profile evolution of transport-limited, Earth Surf. Dynam., 7, 17–43, https://doi.org/10.5194/esurf-7-17-2019, 2019.
Wilkerson, G. V. and Parker, G.: Physical Basis for Quasi-Universal Relationships Describing Bankfull Hydraulic Geometry of Sand-Bed Rivers, J. Hydraul. Eng., 137, 739–753, https://doi.org/10.1061/(ASCE)HY.1943-7900.0000352, 2011.
Womack, W. R. and Schumm, S. A.: Terraces of Douglas Creek, northwestern Colorado: An example of episodic erosion, Geology, 5, 72–76, https://doi.org/10.1130/0091-7613(1977)5<72:TODCNC>2.0.CO;2, 1977.
Young, S. C., Ewing, T., Hamlin, S., Baker, E., and Lupton, D.: Final Report Updating the Hydrogeologic Framework for the Northern Portion of the Gulf Coast Aquifer, Texas Water Development Board, Austin, TX, USA, 283, 2012.
- Abstract
- Introduction
- Geological setting
- Null hypothesis: terrace formation
- Approaches and observations
- Summary of observations
- Discussion and conclusions
- Code availability
- Data availability
- Author contributions
- Competing interests
- Disclaimer
- Acknowledgements
- Financial support
- Review statement
- References
- Supplement
- Abstract
- Introduction
- Geological setting
- Null hypothesis: terrace formation
- Approaches and observations
- Summary of observations
- Discussion and conclusions
- Code availability
- Data availability
- Author contributions
- Competing interests
- Disclaimer
- Acknowledgements
- Financial support
- Review statement
- References
- Supplement