the Creative Commons Attribution 4.0 License.
the Creative Commons Attribution 4.0 License.
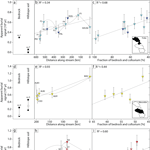
Tracking the 10Be–26Al source-area signal in sediment-routing systems of arid central Australia
John D. Jansen
Toshiyuki Fujioka
Alexandru T. Codilean
David Fink
Réka-Hajnalka Fülöp
Klaus M. Wilcken
David M. Price
Steven Kotevski
L. Keith Fifield
John Chappell
Sediment-routing systems continuously transfer information and mass from eroding source areas to depositional sinks. Understanding how these systems alter environmental signals is critical when it comes to inferring source-area properties from the sedimentary record. We measure cosmogenic 10Be and 26Al along three large sediment-routing systems (∼ 100 000 km2) in central Australia with the aim of tracking downstream variations in 10Be–26Al inventories and identifying the factors responsible for these variations. By comparing 56 new cosmogenic 10Be and 26Al measurements in stream sediments with matching data (n= 55) from source areas, we show that 10Be–26Al inventories in hillslope bedrock and soils set the benchmark for relative downstream modifications. Lithology is the primary determinant of erosion-rate variations in source areas and despite sediment mixing over hundreds of kilometres downstream, a distinct lithological signal is retained. Post-orogenic ranges yield catchment erosion rates of ∼ 6–11 m Myr−1 and silcrete-dominant areas erode as slow as ∼ 0.2 m Myr−1. 10Be–26Al inventories in stream sediments indicate that cumulative-burial terms increase downstream to mostly ∼ 400–800 kyr and up to ∼ 1.1 Myr. The magnitude of the burial signal correlates with increasing sediment cover downstream and reflects assimilation from storages with long exposure histories, such as alluvial fans, desert pavements, alluvial plains, and aeolian dunes. We propose that the tendency for large alluvial rivers to mask their 10Be–26Al source-area signal differs according to geomorphic setting. Signal preservation is favoured by (i) high sediment supply rates, (ii) high mean runoff, and (iii) a thick sedimentary basin pile. Conversely, signal masking prevails in landscapes of (i) low sediment supply and (ii) juxtaposition of sediment storages with notably different exposure histories.
- Article
(7909 KB) - Full-text XML
-
Supplement
(1156 KB) - BibTeX
- EndNote
Landscapes are continuously redistributing mass in response to tectonic and climatic forcing. A suite of surface processes achieves this redistribution at rates fast and slow, modifying landscapes while routing particles from erosional source areas to depositional sinks (Allen, 2008). Rapid, short-term transport (< 101 years) allows for direct monitoring whereas indirect methods such as isotopic tracing or mathematical modelling become necessary beyond historical timescales (> 102 years) (Allen, 2008; Romans et al., 2016). Longer timescales are also relevant to the making of the geological record, which forms the basis of how we understand the narrative of Earth's history (Allen, 2008). The typical approach involves a classic inverse problem whereby attributes of the source area are inferred retrodictively from the geological record. What is inevitably missed, however, is the range of surface processes and dynamics that particles undergo between source and sink. Considering that particles in transit carry an environmental signal of their source area (Romans et al., 2016), this signal is liable to become obscured en route by the intrusion of “noise”, which we take to mean “any modification of the primary signal of interest” (Romans et al., 2016). Indeed, the ratio of signal to noise is the chief limiting factor for accurately inferring source-area information – in addition to the rudimentary understanding of how environmental signals are propagated through sediment-routing systems over > 105 year timescales (Romans et al., 2016).
Modern sediment-routing systems provide the opportunity to track changes in the source-area signal with distance downstream. Arid lowland regions, our focus here, offer insights to the propagation of source-area signals in landscapes of low geomorphic activity. Shield and platform terrain under aridity sustains some of the slowest known erosion rates (Portenga and Bierman, 2011; Struck et al., 2018). These low-relief landscapes are characterised by slow sediment production coupled with slow and intermittent sediment supply to surrounding basins. The typically slow rate of crustal deformation means limited accommodation space, resulting in thin and discontinuous sedimentary records (Armitage et al., 2011). Aridity imposes a strongly episodic character to the sediment-routing system. Infrequent rainfall and stream discharge leads to lengthy and irregular intervals of sediment storage in vast low-gradient river systems. It has been suggested that long hiatuses in sediment transfer may increase the potential for diminishing the signal-to-noise ratio, but this notion is yet to be tested comprehensively.
Terrestrial cosmogenic nuclides are produced by secondary cosmic rays interacting with minerals in the upper few metres of Earth's surface (Gosse and Phillips, 2001); hence they are powerful tools for tracking particle trajectories in the sediment-routing system (Anderson, 2015; Heimsath et al., 2005; Jungers et al., 2009; Matmon et al., 2003; Nichols et al., 2002). Radionuclides, such as 10Be and 26Al, are used widely to quantify the erosional dynamics of landscapes on 103–106 year timescales (Brown et al., 1995; Granger et al., 1996; Lal, 1991; McKean et al., 1993). However, the source-area signal of interest is most often limited to identifying differential erosion rates across a range of spatial scales. For instance, 10Be abundances in bedrock indicate a point-specific weathering rate and in fluvial sediment 10Be is used to derive a spatially averaged catchment erosion rate (Granger et al., 1996). Both approaches entail assumptions that frame how the source-area signal is viewed. Bedrock erosion rate calculations assume steady long-term exhumation (Lal, 1991), and catchment averaging assumes that the fluvial sediment sample is a representative amalgam of particles generated across the entire catchment (Bierman and Steig, 1996; Brown et al., 1995; Granger et al., 1996). Heterogeneity in the sample may arise due to particles sourced disproportionately from (i) faster eroding areas, such as landslides, or (ii) landforms that contain notably longer exposure histories, such as ancient alluvium and aeolian dune fields – either case introduces noise that can bias erosion rate calculations (Granger et al., 1996; Norton et al., 2010). A further key assumption is that samples (including bedrock) have not experienced long-term burial. However, in this case, the noise introduced by burial produces some interesting and exploitable effects. By measuring a nuclide pair with differing radioactive decay rates (e.g. 10Be–26Al) the cumulative burial history can be explicitly tracked by the gradual deviation in the initial production ratio of the two nuclides (Granger and Muzikar, 2001).
Several studies apply this approach to understand how 10Be–26Al source-area signals are modified during transit through the sediment-routing system and suggest two broad limit cases: (i) 10Be–26Al source-area signals remain largely unmodified from source to sink (Clapp et al., 2000, 2001, 2002; Hippe et al., 2012; Wittmann et al., 2011, 2016), or (ii) 10Be–26Al source-area signals become significantly obscured with distance downstream (Bierman et al., 2005; Hidy et al., 2014; Kober et al., 2009). Much remains to be understood about the governing controls on the alteration or otherwise of the source-area signal. The heavy emphasis to date has been with studies of sediment-routing systems conveying a source-area signal specific to rapidly eroding mountain belts (Fig. 1a). It seems likely that the transmission of source-area signals will differ across the much larger proportion of Earth's terrain that is low-relief, tectonically passive, and subject to much lower rates of geomorphic activity (Fig. 1b).
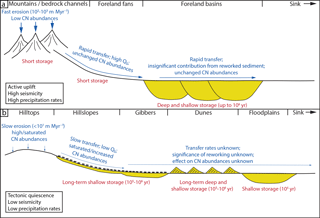
Figure 1Two schematic limit cases of sediment-routing systems (modified after Romans et al., 2016) showing down-system trends from (a) high-relief, tectonically active mountains with a humid climate and (b) a low-relief, post-orogenic setting with an arid climate. Blue script denotes relative rates of erosion and material transfer and their effects on the cosmogenic nuclide inventory (Qs is sediment flux). Red script denotes relative burial depths (shallow < 10 m, deep > 10 m) and storage durations. Yellow shading indicates significant sediment storage.
Here we focus upon the shield and platform landscapes that characterise much of the arid interior of Australia, as well as large portions of other Gondwana segments such as Africa, India, and South America. We measure abundances of cosmogenic 10Be and 26Al in fluvial sediment within rivers draining source areas for which we have established the 10Be–26Al source-area signal from bedrock and hillslope systems (Struck et al., 2018), and we supplement those with four thermoluminescence (TL) ages on floodplain sediments. Tracking the source-area signal through three large sediment-routing systems via a nested set of samples, we investigate (1) downstream variations in source-area 10Be–26Al inventories, (2) the factors that modify the 10Be–26Al source-area signal, and (3) how changes in 10Be–26Al inventories along the course of these streams affect erosion rate calculations. We conclude by reflecting upon the implications of our findings for a source-to-sink understanding of the tempo of change in arid shield–platform landscapes.
Western tributaries of the Eyre Basin: the Finke, Macumba, and Neales rivers drain > 100 000 km2 of the arid continental interior (Fig. 2). Low post-orogenic ranges of early Palaeozoic and Proterozoic rocks (Fig. 3a) and Cenozoic silcrete–duricrust tablelands (Fig. 3b) serve as the major sources of sediment and runoff for the sediment-routing systems. These traverse hundreds of kilometres of low-relief stony soil mantles (Fig. 3c), alluvial plains, and aeolian dune fields before reaching the depositional sink, Lake Eyre (Fig. 1b). The western Eyre Basin experiences mean temperatures of ∼ 20 ∘C and mean rainfall of ∼ 280–130 mm yr−1 with extreme interannual variation. Vegetation is sparse: chenopod shrublands and tussock grasslands predominate in the south and mixed open woodland and spinifex predominate in the north, reflecting the northward transition from winter to summer rainfall dominance (Australian Bureau of Meteorology: http://www.bom.gov.au/climate/, last access: 30 September 2017). Significant flow in the western tributaries is generated mainly by summer rainfall today (Costelloe, 2011; Kotwicki, 1986). Finke River flows have not reached Lake Eyre in historical times (McMahon et al., 2008), but large floods along the Neales have done so repeatedly in more recent years (Kotwicki, 1986; Kotwicki and Isdale, 1991). Periodic high-magnitude flooding in Eyre Basin rivers triggered phases of deposition and incision recorded in fluvial and lacustrine sediments over the last > 300 kyr (Cohen et al., 2012, 2015; Croke et al., 1999; Nanson et al., 1992, 2008).
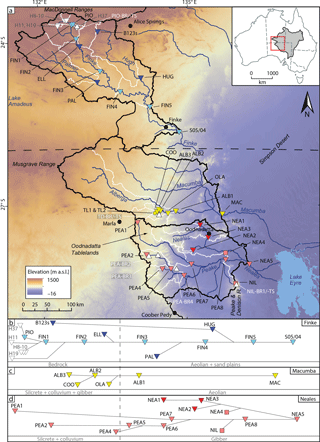
Figure 2(a) Three study catchments in the western Eyre Basin, showing stream sediment samples (downward-pointing triangles and squares), bedrock and hillslope samples (upward-pointing white triangles), and thermoluminescence samples (yellow circle). Finke: trunk stream (light blue) and tributaries (dark blue – this study, white – Heimsath et al., 2010), Macumba (yellow). Neales: Neales subcatchment (dark red triangles), Peake subcatchment (light red triangles), streams draining the Peake and Denison Ranges (light red squares). Eyre Basin (inset: 1.1 million km2) boundaries and outer catchment boundaries (bold black), subcatchment boundaries (white); rivers (blue), towns (black dots), state border (dashed black line). (b, c, d) Schematic sediment-routing networks of the Finke, Macumba, and Neales, subdivided according to overall terrain type.
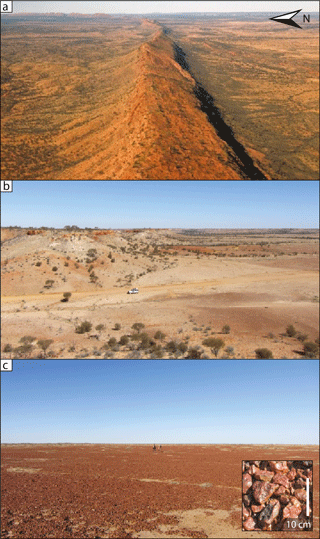
Figure 3(a) Typical strike ridges of steeply inclined strata of the MacDonnell Ranges separated by sediment-mantled terrain, Finke River headwaters (Photo: Geoscience Australia). (b) Flat-topped, silcrete-capped mesas of the Oodnadatta Tablelands, western headwaters of the Neales River (note four-wheel-drive vehicle for scale). (c) Gibber-covered palaeo-alluvial plains in the lower Neales catchment, with distant mesas on the skyline (note persons for scale). Inset shows desert-varnished surface silcrete pebbles.
10Be-derived erosion rates in the Eyre Basin are among the slowest known (Portenga and Bierman, 2011). Rates are < 5–10 m Myr−1 for bedrock outcrops (Fujioka, 2007; Heimsath et al., 2010; Struck et al., 2018) and 5–20 m Myr−1 at the catchment scale (Bierman et al., 1998; Heimsath et al., 2010). The slow evolution of the central Australian landscape is a function of low relief due to restricted tectonic uplift (Jansen et al., 2013; Sandiford, 2002; Sandiford et al., 2009) combined with intensified aridity since the Miocene (Bowler, 1976; Fujioka and Chappell, 2010; Martin, 2006; McGowran et al., 2004). Ongoing intra-plate tectonic deformation is driven by far-field compressive stresses (Hillis et al., 2008; Sandiford and Quigley, 2009; Sandiford et al., 2004; Waclawik et al., 2008) together with dynamic processes beneath the lithosphere, which have caused long-wavelength deformation on the order of hundreds of metres in vertical amplitude (Sandiford et al., 2009). Clear evidence of rapid Neogene to modern uplift occurs on the southern fringe of the Eyre Basin in the Flinders Ranges and at Billa Kalina (Callen and Benbow, 1995; Quigley et al., 2010; Sandiford et al., 2009).
In a comprehensive assessment of 10Be–26Al abundances in bedrock and soil-mantled source areas in the Eyre Basin, Struck et al. (2018) quantify soil residence times of ∼ 0.2–2 Myr and possibly longer at the top of the sediment-routing system. Long residence times and slow hillslope evolution arise from the lack of fluvial incision associated with widespread base-level stability and the long-lasting development of stony soil mantles, also known as desert pavement (Fujioka et al., 2005; Mabbutt, 1977; Matmon et al., 2009; Wells et al., 1995). Hillslope dynamics reflect “top-down” evolution (Montgomery, 2003) with slow rates of authigenic soil production and downslope transport resulting in low connectivity with stream channels (Egholm et al., 2013). Inputs of aeolian dust to soils since at least 0.2 Ma and up to 1 Ma or more lie stabilised beneath stony soil mantles developed over the past ∼ 650 kyr. Nuclide abundances in these source-area materials are naturally very high (Fisher et al., 2014; Fujioka et al., 2005; Struck et al., 2018), but low 26Al ∕ 10Be ratios also suggest a complex history of either cyclic exposure–burial and/or non-steady exhumation on these hillslopes over timescales of 105 to 106 years (Struck et al., 2018).
We set out to test three potential sediment transfer scenarios: (1) 10Be–26Al inventories remain unmodified downstream due to fast (≪ 105 years) sediment transfer and negligible external input; (2) nuclide abundances increase downstream while 26Al ∕ 10Be ratios remain constant, which indicates long-term (≫ 105 years) near-surface particle trajectories, or input from nuclide-rich, burial-free sediment sources; (3) nuclide abundances decrease downstream, suggesting significant radioactive decay during slow sediment transfer with lengthy burial intervals (Granger and Muzikar, 2001; Granger et al., 1996; Schaller et al., 2004) or input from nuclide-poor, long-buried sources.
We used 1 arcsec digital elevation data from the Shuttle Radar Topography Mission (SRTM) to analyse elevation, slope, and mean relief of area upstream of each sediment sample measured for 10Be–26Al (Table 1). Mean catchment relief was calculated via smoothing with a circular kernel of 2.5 km radius. Precipitation data derive from gridded (5 km) mean annual precipitation 1911–2000 (Australian Bureau of Meteorology: http://www.bom.gov.au/climate/, last access: 30 September 2017). Analysis of surface geology is based on a digital 1:1 million surface geology map of Australia (Raymond et al., 2012) and 1 : 250 000 map sheets for additional details. Bedrock and depositional landforms were sorted into seven different classes: exposed bedrock (no silcrete), exposed silcrete, colluvium cover, gibber cover (desert pavement), aeolian cover, sand plains, and alluvium. Of this group, the first three classes were assigned to the bedrock–hillslope domain and the latter four were assigned to the sediment cover domain.
Table 1Catchment characteristics.
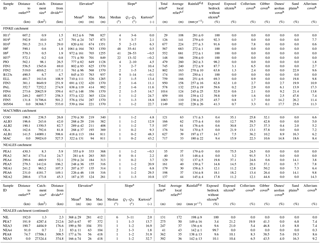
a Based on 1 arcsec SRTM DEM. b Flow distance to most downstream sampling locations derived from watershed delineation in ArcGIS. c Flow distance from drainage divide derived from watershed delineation in ArcGIS. d Uncertainties expressed at 1σ level. e Kurtosis as indicator for the shape of the slope distribution curve and as measure for representativeness of the mean. High kurtosis values indicate pronounced clustering of slope values around the mean. f Catchment average of relief in a 2.5 km radius around every pixel within the catchment. g Based on the average of annual mean precipitation rates between the years 1911 and 2000 (Australian Bureau of Meteorology: http://www.bom.gov.au/climate/, last access: 30 September 2017). h Based on 1:1 million surface geology map of Australia (Raymond et al., 2012). i Samples from Heimsath et al. (2010); labels H8, 9, 10, 11, 19, and 37 (shown in our Fig. 5b and c) refer to MD-108, MD-109, MD-110, MD-111, MD-119, and MD-137 in Heimsath et al. (2010).
3.1 Cosmogenic nuclide analyses
We collected 29 samples of sandy bed material throughout the Finke (n= 11), Macumba (n= 6), and Neales (n= 13) drainage networks (Fig. 2; Table 2) – in addition to 55 10Be and 26Al measurements from bedrock summits and soil mantles in the low-order subcatchments (Struck et al., 2018). Quartz isolation and Be and Al extraction were conducted on the 250–500 µm size fraction of sediment and crushed bedrock samples at the University of Wollongong and at the Australian Nuclear Science and Technology Organisation using standard methods of HF/HNO3 (Kohl and Nishiizumi, 1992), hot phosphoric acid (Mifsud et al., 2013), and ion chromatography (Child et al., 2000). Be and Al isotope ratios were measured on the ANTARES and SIRIUS accelerator mass spectrometers (AMSs) (Fink and Smith, 2007; Wilcken et al., 2017) and normalised to standards KN-5-2 or KN-5-3 (Be) (Nishiizumi et al., 2007) and KN-4-2 (Al) (Nishiizumi, 2004) (Table 2). Uncertainties for the final 10Be and 26Al abundances (Table 2) include AMS measurement uncertainties, 2 % (Be) and 3 % (Al) standard reproducibility, 1 % uncertainty in the Be spike concentration, and 4 % uncertainty in the inductively coupled plasma optical emission spectroscopy (ICP-OES) Al measurements, in quadrature. Erosion rates and apparent burial ages are calculated with CosmoCalc 3.0 (Vermeesch, 2007), using time-independent scaling (Stone, 2000) and production mechanisms based on Granger and Muzikar (2001) to give a sea-level high-latitude (SLHL) spallation production rate for 10Be of 4.18 atoms g−1 y−1 (Vermeesch, 2007). We assume a 10Be half-life of 1.387 ± 0.012 Myr (Chmeleff et al., 2010; Korschinek et al., 2010), 26Al half-life of 0.705 ± 0.024 Myr (Norris et al., 1983), and 26Al ∕ 10Be surface production ratio of 6.75 (Balco et al., 2008). Six samples (UHugh199, UHugh299, UHugh399, UHugh499, Be122p, and Be123s; Table 2) were measured for 10Be at the Australian National University (ANU) Heavy Ion Accelerator Facility (Fifield et al., 2010; see Table 2 for details).
3.2 Thermoluminescence dating
With the aim of gauging the burial age of floodplain sediments flanking some of our study channels, we collected four samples for TL dating in the upper reaches of the Macumba catchment (Fig. 2a): one from a borrow pit at 125 cm depth (TL2-125); the other three (TL1-40, TL1-100, TL1-160) in a depth profile (40, 100, 160 cm depth) from a similar pit close by (Table S1 in the Supplement). All samples were analysed at the University of Wollongong following Shepherd and Price (1990).
Table 2Cosmogenic nuclide data.
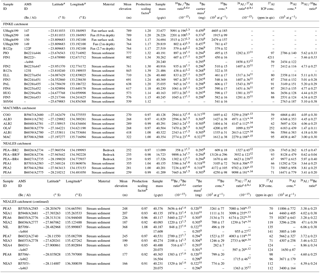
a Coordinates indicate the location of the catchment outlet on the 30 m SRTM DEM; values referenced to WGS84 datum. b Combined atmospheric pressure–latitude scaling factor following the time-independent scaling scheme of Stone (2000). c 10Be ∕ 9Be ratios were normalised to standards. † SRM KN-5-2 (nominal ratio of 8558 × 10−15; 2 % reproducibility error) (Nishiizumi et al., 2007), and ‡ NIST4325 (nominal ratio 27 900 × 10−15; 3 % reproducibility error). d Corrected for batch procedural blanks of 1 1.69 ± 0.92 × 10−15, 2 51.28 ± 7.99 × 10−15, 3 39.26 ± 12.47 × 10−15, 4 7.83 ± 2.10 × 10−15, 5 5.50 ± 0.70 × 10−15, 6 2.94 ± 0.74 × 10−15, and 7 6.24 ± 0.95 × 10−15. e Uncertainties expressed at 1σ level. f Concentrations of 9Be carrier solutions are 8 1090 ± 15 ppm, 9 unknown, 10 1128 ± 22 ppm. g 26Al ∕ 27Al ratios marked with * were blank-corrected using the respective blank's 26Al count rate. h 26Al ∕ 27Al ratios were normalised to SRM KN-4-2 with a nominal ratio of 30,960 × 10−15 (Nishiizumi, 2004). i Corrected for batch procedural blanks of: 11 4.33 ± 1.53 × 10−15, 12 13.57 ± 2.36 × 10−15, 13 10.36 ± 3.76 × 10−15, 14 22.06 ± 5.35 × 10−15, and 15 321.34 ± 25.44 × 10−15. j Samples were excluded from further analyses since 10Be abundances are unnaturally high.
All catchments display low slope gradients overall (≤ 1–3∘), although steeper slopes are rather more common in the Finke (Table 1). Many catchments exhibit a substantial proportion (> 50 %) of bedrock outcrop, especially in the northern Finke strike-ridge country, in the silcrete tablelands in the western Macumba and Neales, and in the Peake and Denison Ranges in the lower Neales catchment. Elsewhere the landscape is draped with a largely continuous cover of stony soil mantles, alluvial plains, and aeolian deposits in varying proportions (Table 1). We use “fraction of bedrock and colluvium” in scatter plots to represent the proportion of source-area terrain upstream of our stream samples (Figs. 4 and 5) – in other words, the area producing the source-area signal that we track downstream through the sediment-routing system.
4.1 10Be abundances in sediment
10Be abundances in stream sediment span 0.3 to 4.3 × 106 atoms g−1 and vary widely among subcatchments (Table 2). Large drainage areas and down-system samples consistently yield 10Be levels at the low end of the range, whereas smaller headwater streams are more variable and tend to span the full range (Fig. 4a). Similarly, relatively low 10Be levels generally follow areas with > 100 m mean relief (almost exclusively within the Finke catchment) and areas of lower relief yield a wide range (Fig. 4b). No relationship exists between 10Be and fraction of bedrock and colluvium in the Finke and Macumba, but high 10Be among the five rocky headwaters of the Peake subcatchment decreases downstream as sediment cover expands (Fig. 4c). These small streams draining the silcrete mesas of the Peake (Fig. 2) yield the highest 10Be levels in stream sediment (Fig. 4). Conversely, the lower Peake receives sediment from the locally steep Peake and Denison Ranges whose small headwater streams yield some of the lowest 10Be in our dataset (Figs. 2 and 4). The effect of such inputs is seen in the low 10Be from the lower Neales samples PEA8 and NEA5 (Figs. 2 and 5h).
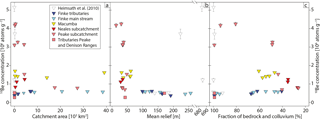
Figure 410Be abundances (normalised to sea-level high latitude) measured in stream sediment relative to (a) drainage area, (b) mean relief, and (c) fraction of exposed bedrock and colluvium cover. Finke samples are blue and white triangles (light blue – trunk stream; dark blue and white – tributaries), Macumba samples are yellow triangles, and Neales samples are red triangles and squares (dark – Neales subcatchment, light – Peake subcatchment, squares – Peake and Denison Ranges).
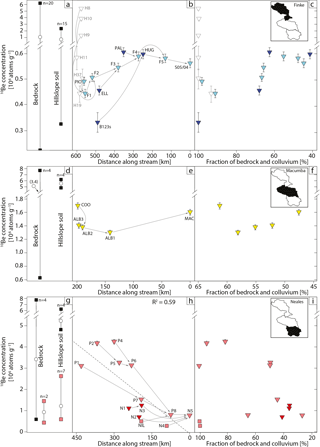
Figure 510Be abundances of bedrock and stream sediment from the Finke (a, b, c) showing trunk streams (light-blue triangles) and tributaries (dark-blue and white triangles), and the Macumba (d, e, f) and Neales (g, h, i) rivers. The Neales data are further subdivided into the subcatchments of Peake (light-red triangles), Neales (dark-red triangles), and Peake and Denison Ranges (light-red squares). Panels (a), (d), and (g) show 10Be abundances in bedrock and hillslope soil as median (open circles) and full range (black squares for MacDonnell Ranges and silcrete, and light-red squares for Peake and Denison Ranges). Panels (b), (e), and (h) show 10Be abundances in stream sediment relative to the distance along-stream from most downstream samples – note that we have reversed the x axes in all panels to illustrate our data from source to sink, left to right. Arrows indicate stream trajectories (sample labels corresponding to Tables: F1-5 are FIN1-5, N1-5 are NEA1-5, and P1-8 are PEA1-8; H denotes samples from Heimsath et al., 2010). Panels (c), (f), and (i), show the fraction of exposed bedrock and colluvium cover. Note that previously published data are included in (a) (Heimsath et al., 2010; Struck et al., 2018) and (d) and (g) (Fujioka et al., 2005; Struck et al., 2018) (see Table S3). All nuclide data are normalised to sea-level high latitude.
4.2 Modelled denudation rates and apparent burial ages in sediment
Overall 26Al ∕ 10Be ratios in sediment span 1.5–6.1, with the majority ∼ 3–5 (20 samples) (Table 2). The Finke displays generally higher 26Al ∕ 10Be ratios (4.7–5.2, interquartile range) relative to the Macumba and Neales (3.5–4.4). Deviation from the steady-state erosion island is typically attributed to one or more episodes of burial–exposure, yet it has been long understood that particle burial cannot be differentiated from non-steady exhumation based on the 26Al ∕ 10Be ratio (Gosse and Phillips, 2001). Hence, we emphasise that our modelled apparent burial ages (Table 3) serve primarily as a measure of deviation from the steady-state erosion curve (Fig. 6). For most of our samples (n= 21) deviations cluster between ∼ 400 and 800 kyr and range up to ∼ 1.1 Myr (Table 3). Low deviations < 400 kyr are exclusively observed in small headwater streams (PIO, FIN1, NEA4, NIL, PEA2), although deviations close to the erosion island are difficult to discriminate due to the spread of uncertainties – the erosion island itself does not accommodate uncertainties in production rate.
Assuming that sediment samples have been continuously exposed at the surface, without decay of nuclides due to burial, the 10Be abundances yield slow catchment-scale denudation rates between 0.3 and 11.0 m Myr−1 (Table 3). When corrected for the “apparent burial age”, as calculated above, denudation rates lower slightly to 0.2–8.1 m Myr−1 (Table 3).
Table 3Basin-wide erosion rates and apparent burial ages.
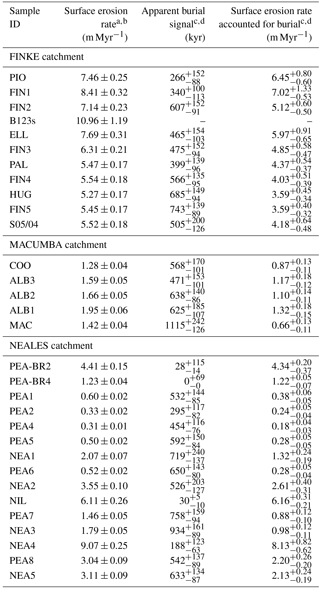
a Calculated from 10Be concentrations with the single-nuclide-erosion tool of CosmoCalc 3.0 (Vermeesch, 2007), using the time-independent scaling scheme of Stone (2000) and production mechanisms based on Granger and Muzikar (2001). b Uncertainties expressed at 1σ level. c Calculated using the CosmoCalc 3.0 (Vermeesch, 2007) burial–erosion tool. The calculation assumes a simple burial scenario, namely, one episode of erosion followed by one episode of burial. The calculation does not account for post-burial re-exposure. d Uncertainties expressed at 1 standard deviation (i.e. 68th percentile).
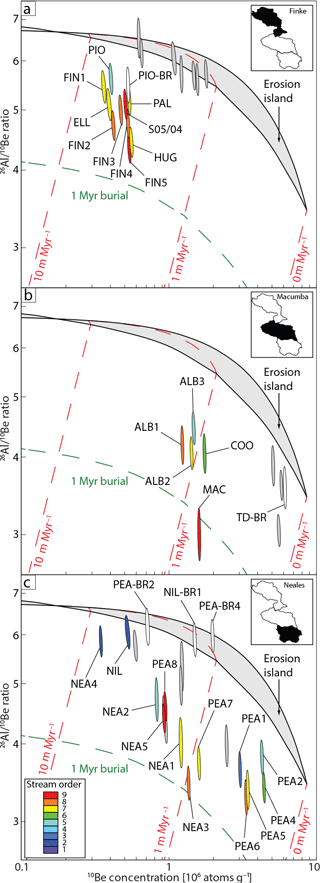
Figure 6Two-nuclide logarithmic plots showing 26Al ∕ 10Be ratios (normalised to sea-level high latitude) in bedrock (white ellipses, “BR”), hillslope soil (grey ellipses), and stream sediments (colour-coded by stream order: low – blue, high – red). (a) Finke catchment; (b) Macumba catchment; (c) Neales catchment. Grey areas represent simple exposure–erosion history (erosion island). Shown are erosion rates (red dashes) and 1 Myr burial isochrons (green). Continuously exposed samples should plot within the steady-state erosion island; samples plotting left of the erosion island indicate a history of post-exhumation burial(s) and/or non-steady exhumation.
5.1 Lithology and the 10Be–26Al source-area signal
10Be levels measured in source-area bedrock and hillslope soil vary widely among our three catchments, but broadly concur within each catchment as reported by Struck et al. (2018) and shown for comparison with samples from the stream network in Fig. 5. Lithology is primarily responsible for the wide variation in erosion rates measured on bedrock surfaces in the western Eyre Basin in the order (from slowest to fastest) silcrete, quartzite, sandstone, and conglomerate (see Fig. 13 in Struck et al., 2018). Compiling bedrock erosion-rate data (n= 26) from Fujioka (2007); Heimsath et al. (2010), and Struck et al. (2018) yields interquartile ranges of 0.2–4.4 m Myr−1 (n= 4) on silcrete mesas in the Oodnadatta Tablelands, 1.6–4.8 m Myr−1 (n= 15) on quartzite–sandstone ridges in the MacDonnell Ranges, 1.8–7.3 m Myr−1 (n= 2) on quartzite–sandstone in the Peake and Denison Ranges, and 6.7–6.8 m Myr−1 (n= 5) on conglomerate in the MacDonnell Ranges. These differences in source-area erosion rates are also reflected in the 10Be levels measured in stream sediments downstream (Fig. 4a), which translate to catchment erosion rates (interquartile ranges) of 4.1–5.8 m Myr−1 in the Finke, 0.9–1.2 m Myr−1 in the Macumba, and 0.3–2.2 m Myr−1 in the Neales. The western headwaters of the Peake yield 0.2–0.4 m Myr−1, which is among the slowest catchment-scale erosion rates ever measured (Table 3).
Our bedrock samples overall have experienced a history of continuous surface exposure or deviate slightly from the steady-state condition (Fig. 6a, c). As proposed by Struck et al. (2018), the minor deviation from the steady-state erosion curve (Fig. 6a) may be the result of non-steady exhumation – termed “two-speed exhumation”. Considering the very low erosion rates (< 1 m Myr−1) we report for the western Eyre Basin, 26Al ∕ 10Be ratios will decrease (< 6.75) throughout the rock column owing to the faster decay of 26Al relative to 10Be. Under these conditions a sudden pulse of erosion due to recent soil-stripping, for instance, will cause surface sample 26Al ∕ 10Be ratios to deviate from the steady-state erosion curve (Fig. 6). Two-speed exhumation provides a viable alternative to cyclic exposure–burial that is usually invoked to account for low 26Al ∕ 10Be ratios (Struck et al., 2018).
5.2 10Be–26Al in the Finke sediment-routing system
The prominent strike ridges and hillslope soil mantles of the MacDonnell Ranges (Fig. 3a) contain a wide range of abundances of 10Be ∼ 0.2–6.5 × 106 atoms g−1 (Fig. 5a), which appears to be driven by bedrock lithology (see Fig. 13 in Struck et al., 2018). In some cases, small alluvial fans form intermediate storages of sediment prior to it entering the stream network, but more commonly bedrock ridges feed sediment directly to low-order headwater streams (Fig. 5b). High 10Be (1–5 × 106 atoms g−1) occurs in streams draining resistant quartzite ridges, whereas streams from sandstone–siltstone ridges and low conglomerate hills yield ∼ 0.3–0.6 × 106 atoms g−1. From the headwaters, 10Be increases slightly over ∼ 300 km downstream (Fig. 5b) to where the channel and floodplain system broadens to unconfined alluvial plains and dune fields (at FIN4, Fig. 2) and from here remains constant downstream. This slight rise in 10Be downstream coincides with the shrinking fraction of bedrock and colluvium (Fig. 5c) and rise in the extent of sediment cover.
The bedrock and soil samples contain a minor burial signal (< 0.3 Myr) (Fig. 7a), which is transmitted to sediments of the headwater streams (Fig. 7b). Similar to the down-system trends in 10Be, the burial signal increases downstream over ∼ 450 km then remains constant (or decreases slightly) to the most downstream sample (Fig. 7b); the apparent burial signal also shows a convincing negative correlation (R2 = 0.68) with the fraction of bedrock and colluvium (Fig. 7c).
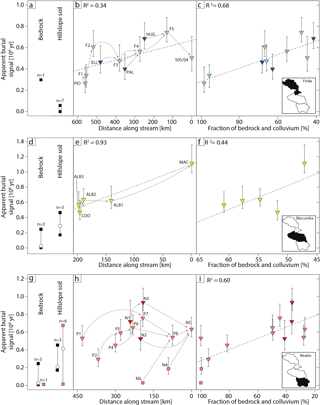
Figure 7Apparent burial ages of bedrock and stream sediment from the Finke (a, b, c) showing trunk streams (light-blue triangles) and tributaries (dark-blue and white triangles), and the Macumba (d, e, f) and Neales (g, h, i) rivers. The Neales data are further subdivided into the subcatchments of Peake (light-red triangles), Neales (dark-red triangles), and the Peake and Denison Ranges (light-red squares). Panels (a), (d), and (g) show apparent burial ages in bedrock and hillslope soil as median (open circles) and full range (black squares for MacDonnell Ranges and silcrete, and light-red squares for Peake and Denison Ranges). Panels (b), (e), and (h) show apparent burial ages in stream sediment relative to the distance along-stream from most downstream samples – note that we have reversed the x axes in all panels to illustrate our data from source to sink, left to right. Arrows indicate stream trajectories (sample labels corresponding to Tables: F1-5 are FIN1-5, N1-5 are NEA1-5, and P1-8 are PEA1-8). Panels (c), (f), and (i) show the fraction of exposed bedrock and colluvium cover.
5.3 10Be–26Al in the Macumba–Neales sediment-routing system
The Macumba and Neales river catchments both drain the silcrete-mesa country of the Oodnadatta Tablelands, which means that their sediment-routing systems share key physiographic and lithological controls. We plot their stream sediment data separately in Figs. 5 and 7, but the bedrock and soil data (Figs. 5d, g and 7d, g) are treated as regionally representative of the Oodnadatta Tablelands.
Silcrete duricrust forms a cap rock that is exceptionally resistant to weathering (Struck et al., 2018) and hence the mesa surfaces tend to accumulate very high 10Be abundances. Based on their work in the Negev, Boroda et al. (2014) propose that the erosion rate of cap rock and mesas scales with their size and extent. Parallel slope retreat, with negligible vertical erosion, predominates on wide tableland plateaus and with ongoing mesa reduction the rate of vertical and horizontal erosion increases to a maximum at the tor stage. Our four samples from silcrete mesas in the Neales and Macumba catchments are intended to represent the full range of bedrock erosion rates (10Be abundances) – starting with a slowly eroding broad plateau (TD-BR, see Struck et al. (2018) for details; ∼ 5.2–7.7 × 106 atoms g−1) to a dissected mesa (PEA-BR4 ∼ 1.7 × 106 atoms g−1) and finally a tor (PEA-BR2 ∼ 0.6 × 106 atoms g−1). The western headwaters of the Neales and Peake subcatchments dissect the eastern edge of a continuous silcrete cap rock plateau (Fig. 2). Given that the degree of mesa dissection increases in the down-system direction (west to east), according to Boroda et al. (2014), we can predict that 10Be supply to the stream network decreases downstream – and this is essentially what we find. Extremely high to rather low 10Be content of mesa bedrock overlaps with data from hillslope soil mantles (Fig. 5g), and the high 10Be accumulated on the flat, un-dissected silcrete plateau is transmitted into the westernmost headwater streams of the Peake subcatchment (Fig. 5h). In contrast, the far more dissected areas drained by the Neales and Macumba headwater streams yield relatively low 10Be (Fig. 5e, h). From the headwaters of the Peake 10Be decreases sharply over ∼ 200–250 km to levels matching the Neales and Macumba streams (Fig. 5h), which both show limited variation over ∼ 200 km downstream (Fig. 5e, h). These downstream trends are broadly accompanied by the reduction in bedrock and expansion of sediment cover (Fig. 5h). The Peake and Denison Ranges in the southeast corner of the Neales catchment (Fig. 2) exerts an important effect on the sediment-routing system. Samples from quartzite–sandstone bedrock together with soil (Fig. 5g) demonstrate that the high relief and weaker lithology is driving erosion rates that are much faster relative to the Oodnadatta Tablelands to the west. Stream sediments from these ranges enter the lower reaches of the Peake and Neales rivers where they notably depress 10Be abundances (Fig. 5h).
The burial signal measured in bedrock and hillslope soil mantles (< 0.6 Myr) is transmitted into headwater streams with fairly similar (or slightly increased) apparent burial ages (Fig. 7d, g). A potential source of low 26Al ∕ 10Be material is generated by fluvial gully heads that undermine the cap rock, yielding deeply shielded (> 3 m) material from beneath the silcrete. The Macumba undergoes a notable increase in burial signal over ∼ 140 km downstream (Fig. 7e), whereas the Neales and Peake subcatchments show a slight increase in burial over ∼ 200 km until this trend is disrupted by inputs from the Peake and Denison Ranges (Fig. 7h). Both the Macumba and Neales networks show a broad increase in burial signal relative to the fraction of sediment cover (Fig. 7f, i).
Cosmogenic nuclide inventories in sediment can be modified in the sediment-routing system via (i) inputs from faster eroding areas or (ii) particles with notably longer exposure histories, including particles buried in transit. We have evidence of the first case in which sediment yield from the faster-eroding Peake and Denison Ranges (Fig. 2) dilutes the high 10Be and depresses the burial signal emanating from the Peake and Neales subcatchments (Figs. 5 and 7). However, the main modification to the 10Be–26Al source-area inventory appears to be the downstream increase in the burial signal (Fig. 7). This modification indicates that samples downstream incorporate a growing fraction of particles derived from temporary storage. Such particles are likely to be a mix of those that have acquired additional nuclides during near-surface (< 1–2 m) exposure to secondary cosmic rays plus those more deeply buried (i.e. > 2–3 m). Only burial can slow down nuclide production, but deep burial is not essential for lowering 26Al ∕ 10Be – even shallow burial can cause deviation from the steady-state erosion curve over timescales on the same order as the 26Al half-life of ∼ 0.7 Myr (see Fig. 14 in Struck et al., 2018). The correlation shown between burial signal and increasing sediment cover (Figs. 7 and 8) is presumably the result of samples assimilating input from storages with long exposure histories that include some (possibly deep) burial. We identify four key sources for such material: (i) alluvial fans, (ii) desert pavements, (iii) floodplains and palaeo-alluvial plains, and (iv) aeolian dunes. Together these landforms span > 50 % of the total catchment area in the lower stream reaches (Figs. 4 and 7; Table 1).
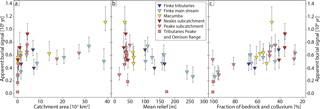
Figure 8Apparent burial ages calculated for stream sediment – using CosmoCalc 3.0 (Vermeesch, 2007) – relative to (a) drainage area, (b) mean relief, and (c) fraction of exposed bedrock and colluvium cover. Finke samples are blue triangles (light blue – trunk stream; dark blue and white – tributaries), Macumba samples are yellow triangles, and Neales samples are red triangles and squares (dark – Neales subcatchment, light – Peake subcatchment, squares – Peake and Denison Ranges).
Alluvial fans are intermediate storages at the transition from hillslopes to the fluvial network; hence they may provide the first opportunity for alteration of the source-area signal. Cosmogenic nuclide depth profiles measured in two typical fans of the upper Finke yield depositional ages of 188–289 ka (Struck et al., 2018) and ∼ 438 to 1474 ka (Fig. S1 in the Supplement). If this is representative of alluvial fans in the region, then we can suggest that alluvial fans play an important role in burial signal development for particles entering headwater streams. Sometimes observed mantling older fans, desert pavement (gibber) occurs throughout the sediment-routing system and nuclide-derived residence times of 105–106 years demonstrate its extreme longevity (Fisher et al., 2014; Fujioka et al., 2005; Struck et al., 2018). Gibbers break off and disperse directly from bedrock outcrop, or they form at the bedrock–soil interface and rise to the surface over time – a process that imparts very low 26Al ∕ 10Be ratios (Struck et al., 2018). Such gibbers released into streams, together with the underlying aeolian soils held in long-term shallow burial, are likely to impact the 10Be–26Al inventory wherever they impinge on channel networks.
The dynamics of sediment transport, temporary storage, and burial are not easy to gauge through fluvial systems that are many hundreds of kilometres long and, in places, tens of kilometres wide (Fig. 2). A few studies link the introduction of a burial signal in modern stream sediment to the reworking of alluvial sediment storages. Kober et al. (2009) suggest that in Rio Lluta, northern Chile, a downstream-increasing burial signal is potentially the result of reworked fluvial terraces (or slope and mass-wasting deposits) up to 105 years old. Similarly, Hidy et al. (2014) find that burial signals in streams on the coastal plain of Texas stem from reworked pre- to mid-Pleistocene deposits. Bierman et al. (2005) identify that reworking long-buried (300–500 kyr) floodplain material produces a burial signal in sediments of Rio Puerco on the Colorado Plateau. Wittmann et al. (2011) detect Amazon floodplain burial signals in coarse (> 500 µm) trunk-stream sediments sourced from reworked storages up to ∼ 1.2 Myr old. In central Australia, some useful guidance to minimum burial duration can be drawn from luminescence ages measured on shallow-buried fluvial sediments. Unlike 10Be–26Al data, which can yield a cumulative burial signal, luminescence burial ages are reset by exposure to sunlight. Previously published TL ages from channel alluvium indicate minimum storage terms of > 200 kyr in the lower Neales (Croke et al., 1996) and > 93 kyr in the lower Finke (Nanson et al., 1995). Our three TL ages (Table S1) from the Macumba River floodplain depth profile increase in age with depth, although the lowermost sample (160 cm) is saturated and therefore may be significantly older than the 120 ± 9 ka from 100 cm depth. Vertical accretion rates at these two floodplain sites span roughly ∼ 8–54 mm kyr−1 and are compatible with the accretion rate of 64 ± 33 mm kyr−1 (mean ±1σ) reported from Cooper Ck floodplain in the eastern Eyre Basin (Jansen et al., 2013). Of the 278 luminescence ages measured in Eyre Basin river sediments, mostly on Cooper Ck, one-third fall between 60 and 120 ka (the oldest being 740 ± 55 ka). Given the climatic and physiographic similarities between the eastern and western Eyre Basin, it seems reasonable to assume that minimum burial durations of > 105 years are representative of the Finke, Macumba, and Neales rivers. If a single storage interval may span ∼ 105 years, then it is feasible that the cumulative effect of many intervals of shallow burial will cause the 26Al ∕ 10Be ratio to deviate.
A similar argument applies to aeolian dune fields, which are major sediment storages spanning ∼ 3 million km2 and up to 40 % of the continent (Hesse, 2010; Wasson et al., 1988). All three catchments of the western Eyre Basin contain dunes in their lower reaches, but the Finke and Macumba have the strongest interaction in their lower reaches fringing the Simpson Desert (Fig. 2). 26Al ∕ 10Be burial ages suggest that dune accumulation probably began up to 1 Myr ago (Fujioka et al., 2009) and, as with alluvial sediments, we infer minimum burial durations from luminescence dating. Based on a recent compilation listing 95 luminescence ages from the Simpson Desert (Hesse, 2016), minimum burial durations of > 105 years are widespread – the oldest dune sample yields a minimum age of 587 ka (Fujioka et al., 2009). In the hyper-arid Namib Desert, Bierman and Caffee (2001) and Vermeesch et al. (2010) suggest that input of aeolian and/or reworked alluvium are responsible for decreased 26Al ∕ 10Be ratios in modern sediments. Similar conclusions are drawn by Davis et al. (2012) for the Nile.
7.1 Lithology drives heterogeneities in the source-area signal
Our comparison of 10Be measured in bedrock outcrops and hillslope soil, with 10Be in headwater streams reiterating the well-known fact that source areas deliver highly diverse 10Be–26Al inventories into stream networks, although the drivers of this diversity are less well understood. In rapidly eroding mountain belts, the wide disparity in source-area erosion rate (102–103 m Myr−1) is typically attributed to the effects of tectonism, such as seismicity and landsliding (Armitage et al., 2011). However, in central Australian streams, a comparable order-of-magnitude spread in source-area erosion rates (10−1–101 m Myr−1) is chiefly due to lithology. Our data show that while 10Be–26Al source-area signals are modified downstream (Fig. 7), disparities in source-area erosion rates remain highly resilient. Despite hundreds of kilometres (∼ 200–600 km) of sediment mixing from source to sink, 10Be–26Al inventories in western Eyre Basin streams (> 1 km2) retain a distinct signal of their source-area lithology (interquartile ranges): 0.2–0.4 m Myr−1 in the upper Peake (silcrete), 0.9–1.2 m Myr−1 in the Macumba (silcrete and granites), and 4.1–5.8 m Myr−1 in the Finke (quartzite–sandstone conglomerate) (Fig. 4a; Table 3). This is consistent with the fundamental role that lithology plays in differentiating the tempo of erosion in all landscapes irrespective of their tectonic or climatic setting (Scharf et al., 2013).
7.2 Are cosmogenic nuclide inventories reliable indicators of source-area erosion rate?
Estimates of catchment-scale erosion rate from cosmogenic nuclide abundances in sediment assume a high-fidelity relationship with the sediment source area (Bierman and Nichols, 2004; Dunai, 2010; Granger and Riebe, 2007; von Blanckenburg, 2005). However, as our data show, the down-system propagation of source-area signals tends to be scale dependent: the widest spread of 10Be occurs among hillslope bedrock outcrops (Fig. 5) from which the buffering effect of sediment transport downslope and downstream leads to progressively more stable catchment-averaged signals of erosion rate or particle burial (Wittmann and von Blanckenburg, 2016). This raises the question of under what circumstances can we expect 10Be–26Al inventories to yield an accurate picture of erosion in the source area. In the western Eyre Basin, the downstream shift in 26Al ∕ 10Be ratio results in erosion-rate disparities (i.e. the difference between upstream and downstream samples) ranging from 2-fold (Finke and Macumba catchments) up to 12-fold (Neales catchment) (Table 3). The validity of the assumption linking 10Be–26Al inventories to their source area reflects a systematic set of geomorphic conditions that requires consideration for reliable erosion rates to be obtained.
Source-area 10Be–26Al inventories are largely unmodified in stream sediments traversing foreland basins fed by tectonically active mountain belts, such as the Andes (Wittmann et al., 2009, 2011), the Alps (Wittmann et al., 2016), and the Himalayas (Lupker et al., 2012; although no 26Al data are available here). Intermediate storage seems to have no appreciable effect on the low-10Be source-area signal conveyed along these large perennial lowland rivers. Their sediment-routing systems are characterised by braiding channels leading on to anabranching and laterally active meandering river styles – all indicative of high-discharge rivers optimised for sediment transfer. Frequent channel avulsion and fast lateral-migration rates bring channels into contact with older floodplain materials, but highly efficient reworking ensures a restricted age spread of sediments within the channel belt and ongoing basin subsidence drives long-term sequestration into a rapidly thickening sediment pile (Allen, 2008; Armitage et al., 2011). In some cases, basin inversion may ultimately lead to recycling of older sediment storages back into the sediment-routing system, as shown in the upper Yellow River where reworked Neogene basin fills alter the 26Al ∕ 10Be source-area ratio downstream (Hu et al., 2011). From these examples, we can infer some key points favouring preservation of source-area signals: (i) high sediment supply rates and therefore a channel–floodplain system configured for high sediment flux, (ii) high mean runoff from headwaters, and (iii) a thick sedimentary basin pile without older basin sediments exposed in the proximal floodplain or terraces.
The alternative limit case, in which the 10Be–26Al source-area signal is modified downstream, follows distinctly different geomorphic conditions, summarised as (i) low sediment supply, and (ii) juxtaposition of sediment storages with notably different exposure histories. Slow rates of source-area erosion (< 20 m Myr−1) typical of low-relief post-orogenic and shield-platform terrain (this study, Bierman et al., 2005; Hidy et al., 2014) produce down-system basin fills that are thin and discontinuous. In the absence of subsidence creating accommodation space, there are juxtaposed sediment storages of widely differing age – and a high prospect of their admixture with the sediment-routing system (Davis et al., 2012; Hidy et al., 2014; Kober et al., 2009). Especially in dryland river systems, atmospheric inputs are typically part of a long-term history of fluvial–aeolian mass exchange (Bierman and Caffee, 2001; Bierman et al., 2005; Davis et al., 2012; Vermeesch et al., 2010). As described above, aeolian dune fields can host particles with notably longer exposure histories and burial timescales > 1 Myr (Fujioka et al., 2009; Vermeesch et al., 2010), and there is much observational evidence of fluvial–aeolian interactions in the western Eyre Basin.
We have tracked downstream variations in 10Be–26Al inventories through three large sediment-routing systems (∼ 100 000 km2) in central Australia by comparing 56 cosmogenic 10Be and 26Al measurements in stream sediments with matching data (n= 55) from bedrock and soil mantles in the headwaters (Struck et al., 2018). Our summary conclusions are as follows.
-
Lithology is the primary determinant of erosion rate variations among bedrock outcrops in the order silcrete, quartzite, sandstone, conglomerate (from slowest to fastest erosion rate). Our regional compilation of bedrock erosion-rate data yields interquartile ranges of 0.2–4.4 m Myr−1 on silcrete mesas in the Oodnadatta Tablelands, 1.6–4.8 m Myr−1 on quartzite–sandstone ridges in the MacDonnell Ranges, 1.8–7.3 m Myr−1 on quartzite–sandstone in the Peake and Denison Ranges, and 6.7–6.8 m Myr−1 on conglomerate in the MacDonnell Ranges. Although 10Be–26Al inventories are modified by sediment mixing over hundreds of kilometres downstream, they still retain a distinct signal of source-area lithology. Sediment-derived catchment-averaged erosion rates (interquartile ranges) are 4.1–5.8 m Myr−1 for the Finke, 0.9–1.2 m Myr−1 for the Macumba, and 0.3–2.2 m Myr−1 for the Neales. The western headwaters of the Peake River (a subcatchment of the Neales River) yield 0.2–0.4 m Myr−1, which is among the slowest catchment-scale erosion rates ever measured (Table 3).
-
10Be–26Al inventories measured in stream-sediment samples from the Finke, Macumba, and Neales rivers all show overall downstream-increasing deviation from the steady-state erosion curve. These deviations correspond to minimum cumulative burial terms mostly between ∼ 400 and 800 kyr (and up to ∼ 1.1 Myr). The magnitude of the burial signal correlates with increasing sediment cover downstream (Figs. 7 and 8) and presumably results from assimilation of shallow-buried sediments from storages with long exposure histories, such as alluvial fans, desert pavements, floodplains and palaeo-alluvial plains, and aeolian dunes. In the lower reaches of the Peake and Neales rivers, the downstream-increasing burial signal is disrupted by inputs from faster-eroding landscapes in the Peake and Denison Ranges.
-
Downstream variations in 10Be–26Al inventories weaken the fidelity of the relationship between source areas and catchment-averaged erosion-rate estimates from samples along large alluvial rivers. Based on our review of case studies that track 10Be–26Al source-area signals downstream, we detect a set of behavioural trends under differing geomorphic settings. Preservation of source-area signals downstream is favoured by (i) high sediment supply rates, (ii) high mean runoff from headwaters, and (iii) a thick sedimentary basin pile without older basin sediments exposed in the proximal floodplain. Conversely, source-area signals are more likely to be modified downstream in landscapes with (i) low sediment supply and (ii) juxtaposition of sediment storages with notably different exposure histories, such as aeolian dune fields. Such modifications can have a significant impact on erosion rate estimates. In desert rivers of the western Eyre Basin, the downstream shift in 26Al ∕ 10Be ratio results in erosion-rate disparities ranging from 2-fold in the Finke and Macumba rivers, and up to 12-fold in the Neales River (Table 3).
All cosmogenic nuclide and thermoluminescence data are available in the tables or in the Supplement. Rainfall data were recorded and provided by the Australian Bureau of Meteorology (http://www.bom.gov.au/jsp/ncc/climate_averages/decadal-rainfall; Australian Bureau of Meteorology, 2017). Lithology data are provided by Geoscience Australia (Raymond et al., 2012; https://data.gov.au/dataset/surface-geology-of-australia-data-package-2012-edition). Elevation data were also provided by Geoscience Australia (https://data.gov.au/dataset/1-second-srtm-derived-hydrological-digital-elevation-model-dem; Geoscience Australia, 2017). Any other data presented and discussed in this article are freely available from Martin Struck (ms646@uowmail.edu.au) or John Jansen (jdj@geo.au.dk).
The supplement related to this article is available online at: https://doi.org/10.5194/esurf-6-329-2018-supplement.
The authors declare that they have no conflict of interest.
We thank Sarah Eccleshall for fieldwork assistance and Charles Mifsud for
assistance with sample processing at ANSTO. Financial support was provided by an Australian
Research Council grant (DP130104023) to Gerald Nanson and John D. Jansen, by a GeoQuEST
Research Centre grant to John D. Jansen and Alexandru T. Codilean, a Marie Skłodowska-Curie Fellowship to
John D. Jansen, and by the Centre for Accelerator Science at ANSTO through the National
Collaborative Research Infrastructure Strategy. Martin Struck received an International
Postgraduate Tuition Award provided by UOW and a matching scholarship funded
by UOW and ANSTO. We acknowledge the Traditional Owners of this country.
Edited by: Jane Willenbring
Reviewed by: two anonymous referees
Allen, P. A.: From landscapes into geological history, Nature, 451, 274–276, https://doi.org/10.1038/nature06586, 2008. a, b, c, d
Anderson, R. S.: Particle trajectories on hillslopes: Implications for particle age and 10Be structure, J. Geophys. Res.-Earth, 120, 1626–1644, https://doi.org/10.1002/2015JF003479, 2015. a
Armitage, J. J., Duller, R. A., Whittaker, A. C., and Allen, P. A.: Transformation of tectonic and climatic signals from source to sedimentary archive, Nat. Geosci., 4, 231–235, https://doi.org/10.1038/ngeo1087, 2011. a, b, c
Australian Bureau of Meteorology: Decadal and multi-decadal mean annual rainfall data, available at: http://www.bom.gov.au/jsp/ncc/climate_averages/decadal-rainfall, last access: 30 September 2017.
Balco, G., Stone, J. O., Lifton, N. A., and Dunai, T. J.: A complete and easily accessible means of calculating surface exposure ages or erosion rates from 10Be and 26Al measurements, Quat. Geochronol., 3, 174–195, https://doi.org/10.1016/j.quageo.2007.12.001, 2008. a
Bierman, P. and Steig, E. J.: Estimating rates of denudation using cosmogenic isotope abundances in sediment, Earth Surf. Proc. Land., 21, 125–139, 1996. a
Bierman, P., Albrecht, A., Bothner, M. H., Brown, E. T., Bullen, D. T., Gray, L. B., and Turpin, L.: Erosion, Weathering, and Sedimentation, in: Isotope Tracers in Catchment Hydrology, edited by: Kendall, C. and McDonnell, J. J., Elsevier, chap. 19, 647–678, https://doi.org/10.1016/B978-0-444-81546-0.50026-4, 1998. a
Bierman, P. R. and Caffee, M.: Slow rates of rock surface erosion and sediment production across the Namib Desert and escarpment, southern Africa, Am. J. Sci., 301, 326–358, https://doi.org/10.2475/ajs.301.4-5.326, 2001. a, b
Bierman, P. R. and Nichols, K. K.: Rock to Sediment-Slope to Sea With 10Be-Rates of Landscape Change, Annu. Rev. Earth Planet. Sci., 32, 215–255, https://doi.org/10.1146/annurev.earth.32.101802.120539, 2004. a
Bierman, P. R., Reuter, J. M., Pavich, M., Gellis, A. C., Caffee, M. W., and Larsen, J.: Using cosmogenic nuclides to contrast rates of erosion and sediment yield in a semi-arid, arroyo-dominated landscape, Rio Puerco Basin, New Mexico, Earth Surf. Proc. Land., 30, 935–953, https://doi.org/10.1002/esp.1255, 2005. a, b, c, d
Boroda, R., Matmon, A., Amit, R., Haviv, I., Arnold, M., Aumaître, G., Bourlès, D. L., Keddadouche, K., Eyal, Y., and Enzel, Y.: Evolution and degradation of flat-top mesas in the hyper-arid Negev, Israel revealed from 10Be cosmogenic nuclides, Earth Surf. Proc. Land., 39, 1611–1621, https://doi.org/10.1002/esp.3551, 2014. a, b
Bowler, J. M.: Aridity in Australia: age, origins and expression in aeolian landforms and sediments, Earth-Sci. Rev., 12, 279–310, https://doi.org/10.1016/0012-8252(76)90008-8, 1976. a
Brown, E. T., Stallard, R. F., Larsen, M. C., Raisbeck, G. M., and Yiou, F.: Denudation rates determined from the accumulation of in situ-produced 10Be in the Luquillo Experimental Forest, Puerto Rico, Earth Planet. Sc. Lett., 129, 193–202, https://doi.org/10.1016/0012-821X(94)00249-X, 1995. a, b
Callen, R. and Benbow, M.: The deserts-Playas, dunefields and watercourses, The Geology of South Australia, 2, 244–251, 1995. a
Child, D., Elliott, G., Mifsud, C., Smith, A., and Fink, D.: Sample processing for earth science studies at ANTARES, Nucl. Instrum. Meth. B, 172, 856–860, https://doi.org/10.1016/S0168-583X(00)00198-1, 2000. a
Chmeleff, J., von Blanckenburg, F., Kossert, K., and Jakob, D.: Determination of the 10Be half-life by multicollector ICP-MS and liquid scintillation counting, Nucl. Instrum. Meth. B, 268, 192–199, https://doi.org/10.1016/j.nimb.2009.09.012, 2010. a
Clapp, E. M., Bierman, P. R., Schick, A. P., Lekach, J., Enzel, Y., and Caffee, M.: Sediment yield exceeds sediment production in arid region drainage basins, Geology, 28, 995–998, https://doi.org/10.1130/0091-7613(2000)28<995:SYESPI>2.0.CO;2, 2000. a
Clapp, E. M., Bierman, P. R., Nichols, K. K., Pavich, M., and Caffee, M.: Rates of sediment supply to arroyos from upland erosion determined using in situ produced cosmogenic 10Be and 26Al, Quaternary Res., 55, 235–245, https://doi.org/10.1006/qres.2000.2211, 2001. a
Clapp, E. M., Bierman, P. R., and Caffee, M.: Using 10Be and 26Al to determine sediment generation rates and identify sediment source areas in an arid region drainage basin, Geomorphology, 45, 89–104, https://doi.org/10.1016/S0169-555X(01)00191-X, 2002. a
Cohen, T. J., Nanson, G. C., Jansen, J. D., Jones, B. G., Jacobs, Z., Larsen, J. R., May, J. H., Treble, P., Price, D. M., and Smith, A. M.: Late Quaternary mega-lakes fed by the northern and southern river systems of central Australia: Varying moisture sources and increased continental aridity, Palaeogeogr. Palaeocl., 356-357, 89–108, https://doi.org/10.1016/j.palaeo.2011.06.023, 2012. a
Cohen, T. J., Jansen, J. D., Gliganic, L. A., Larsen, J. R., Nanson, G. C., May, J.-H., Jones, B. G., and Price, D. M.: Hydrological transformation coincided with megafaunal extinction in central Australia, Geology, 43, 195–198, https://doi.org/10.1130/G36346.1, 2015. a
Costelloe, J.: Hydrological assessment and analysis of the Neales Catchment, A report to the South Australian Arid Lands Natural Resources Management Board, Port Augusta, 2011. a
Croke, J., Magee, J., and Price, D.: Major episodes of Quaternary activity in the lower Neales River, northwest of Lake Eyre, central Australia, Palaeogeogr. Palaeocl., 124, 1–15, https://doi.org/10.1016/0031-0182(96)00016-8, 1996. a
Croke, J., Magee, J., and Wallensky, E.: The role of the Australian Monsoon in the western catchment of Lake Eyre, central Australia, during the Last Interglacial, Quatern. Int., 57, 71–80, https://doi.org/10.1016/S1040-6182(98)00051-2, 1999. a
Davis, M., Matmon, A., Rood, D. H., and Avnaim-Katav, S.: Constant cosmogenic nuclide concentrations in sand supplied from the Nile River over the past 2.5 my, Geology, 40, 359–362, https://doi.org/10.1130/G32574.1, 2012. a, b, c
Dunai, T. J.: Cosmogenic nuclides: principles, concepts and applications in the earth surface sciences, Cambridge University Press, 2010. a
Egholm, D. L., Knudsen, M. F., and Sandiford, M.: Lifespan of mountain ranges scaled by feedbacks between landsliding and erosion by rivers, Nature, 498, 475–8, https://doi.org/10.1038/nature12218, 2013. a
Fifield, L. K., Tims, S., Fujioka, T., Hoo, W. T., and Everett, S.: Accelerator mass spectrometry with the 14UD accelerator at the Australian National University, Nucl. Instrum. Meth. B, 268, 858–862, https://doi.org/10.1016/j.nimb.2009.10.049, 2010. a
Fink, D. and Smith, A.: An inter-comparison of 10Be and 26Al AMS reference standards and the 10Be half-life, Nucl. Instrum. Meth. B, 259, 600–609, https://doi.org/10.1016/j.nimb.2007.01.299, 2007. a
Fisher, A., Fink, D., Chappell, J., and Melville, M.: 26Al/10Be dating of an aeolian dust mantle soil in western New South Wales, Australia, Geomorphology, 219, 201–212, https://doi.org/10.1016/j.geomorph.2014.05.007, 2014. a, b
Fujioka, T.: Development of in situ cosmogenic 21Ne exposure dating, and dating of Australian arid landforms by combined stable and radioactive in situ cosmogenic nuclides, PhD Thesis, The Australian National University, Canberra, Australia, 2007. a, b
Fujioka, T. and Chappell, J.: History of Australian aridity: chronology in the evolution of arid landscapes, Geological Society, London, Special Publications, 346, 121–139, https://doi.org/10.1144/sp346.8, 2010. a
Fujioka, T., Chappell, J., Honda, M., Yatsevich, I., Fifield, K., and Fabel, D.: Global cooling initiated stony deserts in central Australia 2–4 Ma, dated by cosmogenic 21Ne-10Be, Geology, 33, 993–996, https://doi.org/10.1130/g21746.1, 2005. a, b, c, d
Fujioka, T., Chappell, J., Fifield, L. K., and Rhodes, E. J.: Australian desert dune fields initiated with Pliocene-Pleistocene global climatic shift, Geology, 37, 51–54, https://doi.org/10.1130/g25042a.1, 2009. a, b, c
Geoscience Australia, 1 second SRTM Derived Hydrological Digital Elevation Model (DEM-H) version 1.0, available at: https://data.gov.au/dataset/1-second-srtm-derived-hydrological-digital-elevation-model-dem, last access: 30 September 2017.
Gosse, J. C. and Phillips, F. M.: Terrestrial in situ cosmogenic nuclides: theory and application, Quaternary Sci. Rev., 20, 1475–1560, https://doi.org/10.1016/S0277-3791(00)00171-2, 2001. a, b
Granger, D. and Riebe, C.: Cosmogenic nuclides in weathering and erosion, Treatise on geochemistry, 5, 1–43, 2007. a
Granger, D. E. and Muzikar, P. F.: Dating sediment burial with in situ-produced cosmogenic nuclides: theory, techniques, and limitations, Earth Planet. Sc. Lett., 188, 269–281, https://doi.org/10.1016/S0012-821X(01)00309-0, 2001. a, b, c, d
Granger, D. E., Kirchner, J. W., and Finkel, R.: Spatially averaged long-term erosion rates measured from in situ-produced cosmogenic nuclides in alluvial sediment, J. Geol., 104, 249–257, https://doi.org/10.1086/629823, 1996. a, b, c, d, e
Heimsath, A. M., Furbish, D. J., and Dietrich, W. E.: The illusion of diffusion: Field evidence for depth-dependent sediment transport, Geology, 33, 949–952, https://doi.org/10.1130/g21868.1, 2005. a
Heimsath, A. M., Chappell, J., and Fifield, K.: Eroding Australia: rates and processes from Bega Valley to Arnhem Land, Geological Society, London, Special Publications, 346, 225–241, https://doi.org/10.1144/sp346.12, 2010. a, b, c, d, e, f, g, h
Hesse, P. P.: The Australian desert dunefields: formation and evolution in an old, flat, dry continent, Geological Society, London, Special Publications, 346, 141–164, https://doi.org/10.1144/sp346.9, 2010. a
Hesse, P. P.: How do longitudinal dunes respond to climate forcing? Insights from 25 years of luminescence dating of the Australian desert dunefields, Quatern. Int., 410, 11–29, https://doi.org/10.1016/j.quaint.2014.02.020, 2016. a
Hidy, A. J., Gosse, J. C., Blum, M. D., and Gibling, M. R.: Glacial–interglacial variation in denudation rates from interior Texas, USA, established with cosmogenic nuclides, Earth Planet. Sc. Lett., 390, 209–221, https://doi.org/10.1016/j.epsl.2014.01.011, 2014. a, b, c, d
Hillis, R. R., Sandiford, M., Reynolds, S. D., and Quigley, M. C.: Present-day stresses, seismicity and Neogene-to-Recent tectonics of Australia's “passive” margins: intraplate deformation controlled by plate boundary forces, Geological Society, London, Special Publications, 306, 71–90, https://doi.org/10.1144/SP306.3, 2008. a
Hippe, K., Kober, F., Zeilinger, G., Ivy-Ochs, S., Maden, C., Wacker, L., Kubik, P. W., and Wieler, R.: Quantifying denudation rates and sediment storage on the eastern Altiplano, Bolivia, using cosmogenic 10Be, 26Al, and in situ 14C, Geomorphology, 179, 58–70, https://doi.org/10.1016/j.geomorph.2012.07.031, 2012. a
Hu, X., Kirby, E., Pan, B., Granger, D. E., and Su, H.: Cosmogenic burial ages reveal sediment reservoir dynamics along the Yellow River, China, Geology, 39, 839–842, https://doi.org/10.1130/G32030.1, 2011. a
Jansen, J. D., Nanson, G. C., Cohen, T. J., Fujioka, T., Fabel, D., Larsen, J. R., Codilean, A. T., Price, D. M., Bowman, H. H., May, J. H., and Gliganic, L. A.: Lowland river responses to intraplate tectonism and climate forcing quantified with luminescence and cosmogenic 10Be, Earth Planet. Sc. Lett., 366, 49–58, https://doi.org/10.1016/j.epsl.2013.02.007, 2013. a, b
Jungers, M. C., Bierman, P. R., Matmon, A., Nichols, K., Larsen, J., and Finkel, R.: Tracing hillslope sediment production and transport with in situ and meteoric 10Be, J. Geophys. Res.-Earth, 114, F04020, https://doi.org/10.1029/2008JF001086, 2009. a
Kober, F., Ivy-Ochs, S., Zeilinger, G., Schlunegger, F., Kubik, P. W., Baur, H., and Wieler, R.: Complex multiple cosmogenic nuclide concentration and histories in the arid Rio Lluta catchment, northern Chile, Earth Surf. Proc. Land., 34, 398–412, https://doi.org/10.1002/esp.1748, 2009. a, b, c
Kohl, C. and Nishiizumi, K.: Chemical isolation of quartz for measurement of in-situ-produced cosmogenic nuclides, Geochim. Cosmochim. Ac., 56, 3583–3587, https://doi.org/10.1016/0016-7037(92)90401-4, 1992. a
Korschinek, G., Bergmaier, A., Faestermann, T., Gerstmann, U., Knie, K., Rugel, G., Wallner, A., Dillmann, I., Dollinger, G., Von Gostomski, C. L., Kossert, K., Maiti, M., Poutivtsev, M., and Remmert, A.: A new value for the half-life of 10Be by heavy-ion elastic recoil detection and liquid scintillation counting, Nucl. Instrum. Meth. B, 268, 187–191, https://doi.org/10.1016/j.nimb.2009.09.020, 2010. a
Kotwicki, V.: Floods of Lake Eyre, Adelaide (Australia) Engineering and Water Supply Dept., 1986. a, b
Kotwicki, V. and Isdale, P.: Hydrology of Lake Eyre, Australia: El Nino link, Palaeogeogr. Palaeocl., 84, 87–98, https://doi.org/10.1016/0031-0182(91)90037-R, 1991. a
Lal, D.: Cosmic ray labeling of erosion surfaces: in situ nuclide production rates and erosion models, Earth Planet. Sc. Lett., 104, 424–439, https://doi.org/10.1016/0012-821X(91)90220-C, 1991. a, b
Lupker, M., Blard, P.-H., Lavé, J., France-Lanord, C., Leanni, L., Puchol, N., Charreau, J., and Bourlès, D.: 10Be-derived Himalayan denudation rates and sediment budgets in the Ganga basin, Earth Planet. Sc. Lett., 333–334, 146–156, https://doi.org/10.1016/j.epsl.2012.04.020, 2012. a
Mabbutt, J. A.: Desert landforms, Australian National University Press, Canberra, 1977. a
Martin, H.: Cenozoic climatic change and the development of the arid vegetation in Australia, J. Arid Environ., 66, 533–563, https://doi.org/10.1016/j.jaridenv.2006.01.009, 2006. a
Matmon, A., Bierman, P., Larsen, J., Southworth, S., Pavich, M., and Caffee, M.: Temporally and spatially uniform rates of erosion in the southern Appalachian Great Smoky Mountains, Geology, 31, 155–158, 2003. a
Matmon, A., Simhai, O., Amit, R., Haviv, I., Porat, N., McDonald, E., Benedetti, L., and Finkel, R.: Desert pavement-coated surfaces in extreme deserts present the longest-lived landforms on Earth, Geol. Soc. Am. Bull., 121, 688–697, https://doi.org/10.1130/b26422.1, 2009. a
McGowran, B., Holdgate, G., Li, Q., and Gallagher, S.: Cenozoic stratigraphic succession in southeastern Australia, Aust. J. Earth Sci., 51, 459–496, https://doi.org/10.1111/j.1400-0952.2004.01078.x, 2004. a
McKean, J. A., Dietrich, W. E., Finkel, R. C., Southon, J. R., and Caffee, M. W.: Quantification of soil production and downslope creep rates from cosmogenic 10Be accumulations on a hillslope profile, Geology, 21, 343–346, https://doi.org/10.1130/0091-7613(1993)021<0343:QOSPAD>2.3.CO;2, 1993. a
McMahon, T. A., Murphy, R. E., Peel, M. C., Costelloe, J. F., and Chiew, F. H. S.: Understanding the surface hydrology of the Lake Eyre Basin: Part 1 – Rainfall, J. Arid Environ., 72, 1853–1868, https://doi.org/10.1016/j.jaridenv.2008.06.004, 2008. a
Mifsud, C., Fujioka, T., and Fink, D.: Extraction and purification of quartz in rock using hot phosphoric acid for in situ cosmogenic exposure dating, Nucl. Instrum. Meth. B, 294, 203–207, https://doi.org/10.1016/j.nimb.2012.08.037, 2013. a
Montgomery, D. R.: Predicting landscape-scale erosion rates using digital elevation models, C. R. Geosci., 335, 1121–1130, https://doi.org/10.1016/j.crte.2003.10.005, 2003. a
Nanson, G., Chen, X., and Price, D.: Aeolian and fluvial evidence of changing climate and wind patterns during the past 100 ka in the western Simpson Desert, Australia, Palaeogeogr. Palaeocl., 113, 87–102, https://doi.org/10.1016/0031-0182(95)00064-S, 1995. a
Nanson, G. C., Price, D. M., and Short, S. A.: Wetting and drying of Australia over the past 300 ka, Geology, 20, 791–794, https://doi.org/10.1130/0091-7613(1992)020<0791:WADOAO>2.3.CO;2, 1992. a
Nanson, G. C., Price, D. M., Jones, B. G., Maroulis, J. C., Coleman, M., Bowman, H., Cohen, T. J., Pietsch, T. J., and Larsen, J. R.: Alluvial evidence for major climate and flow regime changes during the middle and late Quaternary in eastern central Australia, Geomorphology, 101, 109–129, https://doi.org/10.1016/j.geomorph.2008.05.032, 2008. a
Nichols, K. K., Bierman, P. R., Hooke, R. L., Clapp, E. M., and Caffee, M.: Quantifying sediment transport on desert piedmonts using 10Be and 26Al, Geomorphology, 45, 105–125, https://doi.org/10.1016/S0169-555X(01)00192-1, 2002. a
Nishiizumi, K.: Preparation of 26Al AMS standards, Nucl. Instrum. Meth. B, 223, 388–392, https://doi.org/10.1016/j.nimb.2004.04.075, 2004. a, b
Nishiizumi, K., Imamura, M., Caffee, M. W., Southon, J. R., Finkel, R. C., and McAninch, J.: Absolute calibration of 10Be AMS standards, Nucl. Instrum. Meth. B, 258, 403–413, https://doi.org/10.1016/j.nimb.2007.01.297, 2007. a, b
Norris, T., Gancarz, A., Rokop, D., and Thomas, K.: Half-life of 26Al, in: Lunar and planetary science conference proceedings, 14, B331–B333, https://doi.org/10.1029/JB088iS01p0B331, 1983. a
Norton, K. P., von Blanckenburg, F., and Kubik, P. W.: Cosmogenic nuclide-derived rates of diffusive and episodic erosion in the glacially sculpted upper Rhone Valley, Swiss Alps, Earth Surf. Proc. Land., 35, 651–662, https://doi.org/10.1002/esp.1961, 2010. a
Portenga, E. W. and Bierman, P. R.: Understanding Earth's eroding surface with 10Be, GSA Today, 21, 4–10, https://doi.org/10.1130/g111a.1, 2011. a, b
Quigley, M. C., Clark, D., and Sandiford, M.: Tectonic geomorphology of Australia, Geological Society, London, Special Publications, 346, 243–265, https://doi.org/10.1144/sp346.13, 2010. a
Raymond, O., Liu, S., Gallagher, R., Zhang, W., and Highet, L.: Surface Geology of Australia 1:1 million scale (2012 Edn.), Commonwealth of Australia (Geoscience Australia), available at: https://data.gov.au/dataset/surface-geology-of-australia-data-package-2012-edition (last access: 30 September 2017) 2012. a, b
Romans, B. W., Castelltort, S., Covault, J. A., Fildani, A., and Walsh, J.: Environmental signal propagation in sedimentary systems across timescales, Earth-Sci. Rev., 153, 7–29, https://doi.org/10.1016/j.earscirev.2015.07.012, 2016. a, b, c, d, e
Sandiford, M.: Low thermal Peclet number intraplate orogeny in central Australia, Earth Planet. Sc. Lett., 201, 309–320, https://doi.org/10.1016/S0012-821X(02)00723-9, 2002. a
Sandiford, M. and Quigley, M.: TOPO-OZ: Insights into the various modes of intraplate deformation in the Australian continent, Tectonophysics, 474, 405–416, https://doi.org/10.1016/j.tecto.2009.01.028, 2009. a
Sandiford, M., Wallace, M., and Coblentz, D.: Origin of the in situ stress field in south-eastern Australia, Basin Res., 16, 325–338, https://doi.org/10.1111/j.1365-2117.2004.00235.x, 2004. a
Sandiford, M., Quigley, M., de Broekert, P., and Jakica, S.: Tectonic framework for the Cenozoic cratonic basins of Australia, Aust. J. Earth Sci., 56, S5–S18, https://doi.org/10.1080/08120090902870764, 2009. a, b, c
Schaller, M., Blanckenburg, F. v., Hovius, N., Veldkamp, A., van den Berg, M. W., and Kubik, P.: Paleoerosion rates from cosmogenic 10Be in a 1.3 Ma terrace sequence: response of the River Meuse to changes in climate and rock uplift, J. Geol., 112, 127–144, https://doi.org/10.1086/381654, 2004. a
Scharf, T. E., Codilean, A. T., de Wit, M., Jansen, J. D., and Kubik, P. W.: Strong rocks sustain ancient postorogenic topography in southern Africa, Geology, 41, 331–334, https://doi.org/10.1130/g33806.1, 2013. a
Shepherd, M. and Price, D.: Thermoluminescence dating of late Quaternary dune sand, Manawatu/Horowhenua area, New Zealand: a comparison with 14C age determinations, New Zeal. J. Geol. Geop., 33, 535–539, https://doi.org/10.1080/00288306.1990.10421371, 1990. a
Stone, J. O.: Air pressure and cosmogenic isotope production, J. Geophys. Res., 105, 23753, https://doi.org/10.1029/2000jb900181, 2000. a, b, c
Struck, M., Jansen, J. D., Fujioka, T., Codilean, A. T., Fink, D., Egholm, D. L., Fülöp, R.-H., Wilcken, K. M., and Kotevski, S.: Soil production and transport on postorogenic desert hillslopes quantified with 10Be and 26Al, Geol. Soc. Am. Bull., 130, 1017–1040, https://doi.org/10.1130/B31767.1, 2018. a, b, c, d, e, f, g, h, i, j, k, l, m, n, o, p, q, r, s, t, u
Vermeesch, P.: CosmoCalc: An Excel add-in for cosmogenic nuclide calculations, Geochem. Geophys. Geosys., 8, https://doi.org/10.1029/2006GC001530, 2007. a, b, c, d, e
Vermeesch, P., Fenton, C., Kober, F., Wiggs, G., Bristow, C. S., and Xu, S.: Sand residence times of one million years in the Namib Sand Sea from cosmogenic nuclides, Nat. Geosci., 3, 862–865, https://doi.org/10.1038/ngeo985, 2010. a, b, c
von Blanckenburg, F.: The control mechanisms of erosion and weathering at basin scale from cosmogenic nuclides in river sediment, Earth Planet. Sc. Lett., 237, 462–479, https://doi.org/10.1016/j.epsl.2005.06.030, 2005. a
Waclawik, V. G., Lang, S. C., and Krapf, C. B. E.: Fluvial response to tectonic activity in an intra-continental dryland setting: The Neales River, Lake Eyre, Central Australia, Geomorphology, 102, 179–188, https://doi.org/10.1016/j.geomorph.2007.06.021, 2008. a
Wasson, R. J., Fitchett, K., Mackey, B., and Hyde, R.: Large-scale patterns of dune type, spacing and orientation in the Australian continental dunefield, Aust. Geogr., 19, 89–104, https://doi.org/10.1080/00049188808702952, 1988. a
Wells, S. G., McFadden, L. D., Poths, J., and Olinger, C. T.: Cosmogenic 3He surface-exposure dating of stone pavements: Implications for landscape evolution in deserts, Geology, 23, 613–616, https://doi.org/10.1130/0091-7613(1995)023<0613:CHSEDO>2.3.CO;2, 1995. a
Wilcken, K., Fink, D., Hotchkis, M., Garton, D., Button, D., Mann, M., Kitchen, R., Hauser, T., and O´Connor, A.: Accelerator Mass Spectrometry on SIRIUS: New 6MV spectrometer at ANSTO, Nucl. Instrum. Meth. B, 1, 278–282, https://doi.org/10.1016/j.nimb.2017.01.003, 2017. a
Wittmann, H. and von Blanckenburg, F.: The geological significance of cosmogenic nuclides in large lowland river basins, Earth-Sci. Rev., 159, 118–141, https://doi.org/10.1016/j.earscirev.2016.06.001, 2016. a
Wittmann, H., von Blanckenburg, F., Guyot, J. L., Maurice, L., and Kubik, P. W.: From source to sink: Preserving the cosmogenic 10Be-derived denudation rate signal of the Bolivian Andes in sediment of the Beni and Mamoré foreland basins, Earth Planet. Sc. Lett., 288, 463–474, https://doi.org/10.1016/j.epsl.2009.10.008, 2009. a
Wittmann, H., von Blanckenburg, F., Maurice, L., Guyot, J. L., and Kubik, P. W.: Recycling of Amazon floodplain sediment quantified by cosmogenic 26Al and 10Be, Geology, 39, 467–470, https://doi.org/10.1130/g31829.1, 2011. a, b, c
Wittmann, H., Malusà, M. G., Resentini, A., Garzanti, E., and Niedermann, S.: The cosmogenic record of mountain erosion transmitted across a foreland basin: Source-to-sink analysis of in situ 10Be, 26Al and 21Ne in sediment of the Po river catchment, Earth Planet. Sc. Lett., 452, 258–271, https://doi.org/10.1016/j.epsl.2016.07.017, 2016. a, b
- Abstract
- Introduction
- Sediment-routing and timescales of landscape evolution in central Australia
- Methods
- Results
- Down-system variation in 10Be–26Al in the western Eyre Basin
- Factors that modify the 10Be–26Al source-area signal
- The 10Be–26Al source-area signal in sediment-routing systems – a synthesis
- Conclusions
- Data availability
- Competing interests
- Acknowledgements
- References
- Supplement
- Abstract
- Introduction
- Sediment-routing and timescales of landscape evolution in central Australia
- Methods
- Results
- Down-system variation in 10Be–26Al in the western Eyre Basin
- Factors that modify the 10Be–26Al source-area signal
- The 10Be–26Al source-area signal in sediment-routing systems – a synthesis
- Conclusions
- Data availability
- Competing interests
- Acknowledgements
- References
- Supplement