the Creative Commons Attribution 4.0 License.
the Creative Commons Attribution 4.0 License.
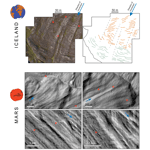
Long-runout landslides with associated longitudinal ridges in Iceland as analogues of Martian landslide deposits
Giulia Magnarini
Anya Champagne
Costanza Morino
Calvin Beck
Meven Philippe
Armelle Decaulne
Susan J. Conway
Much work has been done to study the behaviour of long-runout landslides and their associated longitudinal ridges, yet the origin of the hypermobility of such landslides and the formation mechanism of longitudinal ridges are poorly understood. As terrestrial long-runout landslides emplaced on glaciers commonly exhibit longitudinal ridges, the presence of these landforms has been used to infer the presence of ice on Mars, where hundreds of well-preserved long-runout landslides with longitudinal ridges are found. However, the presence of the same landforms in regions where extensive glaciations did not occur, for instance, on the Moon and in the Atacama region on Earth, suggests that ice is not the only factor influencing the formation of long-runout landslides with longitudinal ridges.
Iceland is a unique region for its high spatial density of well-preserved long-runout landslides with longitudinal ridges. Here, we compiled the first catalogue of Icelandic long-runout landslides with longitudinal ridges, and we compared them with Martian long-runout landslides with longitudinal ridges of similar length. Moreover, we present detailed morphological observations of the Dalvík landslide deposit, in the Tröllaskagi peninsula, Iceland, and compare them with morphological observations of Martian landslides.
Our results show that Icelandic long-runout landslides share key features with Martian analogue deposits, including splitting of longitudinal ridges and development of associated en echelon features. Therefore, Icelandic long-runout landslides with longitudinal ridges represent good morphological analogues of Martian long-runout landslides. Moreover, Iceland offers an opportunity to investigate the occurrence of these landforms at a regional scale, as well as their link with deglaciation following the Last Glacial Maximum, which could also provide insights into Martian palaeoclimatic and palaeoenvironmental conditions.
- Article
(17640 KB) - Full-text XML
-
Supplement
(15017 KB) - BibTeX
- EndNote
Long-runout landslides are hypermobile landslides that can have distinctive morphologies, such as raised terminal edges, lateral levees, and longitudinal ridges. Longitudinal ridges are features visible at the surface of the deposit and extend parallel to the direction of movement (Fig. 1). The hypermobility of long-runout landslides is expressed by their horizontal runouts (L) that are significantly longer than their vertical drops (H). In the literature, the ratio is used to describe the mobility of landslides, which for long-runout landslides is significantly lower than 0.6 (e.g. Heim, 1932; Hsü, 1978; Legros, 2002). Therefore, the definition of a long-runout landslide is not solely based on the final horizontal length of the deposit. In fact, the final horizontal length of long-runout landslides can range across 2 orders of magnitude, from shorter than 1 km to tens of kilometres (Legros, 2002, and references within). However, it appears that a volume threshold (106 m3) exists, below which landslides do not develop a long-runout (e.g. Heim, 1932; Legros, 2002).
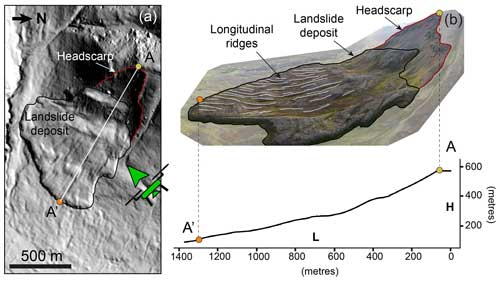
Figure 1Example of a terrestrial long-runout landslide with longitudinal ridges. The red line shows the headscarp of the landslide. The black line shows the extent of the landslide deposit. The white lines in panel (b) show the longitudinal ridges that mark the landslide deposit and extend parallel to the direction of the movement. The yellow and orange dots represent the highest point of the headscarp and the lowest point of the deposit, respectively; these locations are used to derive the elevation drop (H) and the horizontal extent (L) of the landslide along the topographic profile obtained along the section A–A′ (white line in panel a). Panel (a) shows the hillshade model of the Dalvík landslide obtained from the 2 m px−1 resolution ArcticDEM (Porter et al., 2018). Panel (b) shows a photomosaic from drone imagery of the Dalvík landslide and its longitudinal topographic profile. The green arrowhead in panel (a) shows the view direction of the images acquired with the drone and used to generate the photomosaic in panel (b).
Long-runout landslides and their associated longitudinal ridges are common in the solar system (Beddingfield et al., 2020; Lucchitta, 1979; Schmidt et al., 2017; Singer et al., 2012), and their behaviour has been extensively studied (e.g. Davies and McSaveney, 2012; Harrison and Grimm, 2003; Legros, 2002; Lucas et al., 2014; Pudasaini and Miller, 2013; Vardoulakis, 2000; Voight and Faust, 1982), yet the origin of the hypermobility of such landslides and the formation mechanism of the longitudinal ridges are poorly understood. So far, two hypotheses have emerged: (1) the environment-dependent origin, in which environmental conditions, namely the presence of basal ice (De Blasio, 2011, 2014; Dufresne and Davies, 2009; Lucchitta, 1987; Mège and Bourgeois, 2011; Schmidt et al., 2017) and/or clays (Watkins et al., 2015), is a necessary condition for the development of long-runout landslides and their associated longitudinal ridges. According to this hypothesis, low-friction surfaces would favour tensional deformation of the sliding mass by both longitudinal stretching and lateral spreading (De Blasio, 2011; Dufresne and Davies, 2009). Such tensile deformation would generate the longitudinal structures observed on the surface of long-runout landslide deposits by the mechanism of necking, similar to the tensile deformation that generates boudinage in tectonic contexts. The second hypothesis is (2) the environment-independent origin, in which the development of longitudinal ridges does not depend on the presence of a specific environmental condition and/or lithology (Magnarini et al., 2019, 2021a, b). Magnarini et al. (2019) propose that longitudinal ridges that characterise terrestrial and planetary long-runout landslides may be the expression of a mechanical instability that emerges within the flowing mass once a velocity threshold is surpassed, as observed in laboratory experiments on rapid granular flows (Forterre and Pouliquen, 2001). However, as this mechanism involves the generation of helicoidal convection cells within the moving landslide, it is contradicted by the evidence of the preservation of the original stratigraphic layering within the final deposit that is attributed to the lack of turbulence during the emplacement of long-runout landslides (e.g. Dufresne et al., 2016; Johnson, 1978; Magnarini et al., 2021a; Shreve, 1968; Weidinger et al., 2014). To reconcile with field observations, Magnarini et al. (2021a) speculate on the existence of a vibration-assisted mechanism that would be able to produce the longitudinal pattern via mechanical instability yet maintain the internal structures.
As terrestrial long-runout landslides emplaced on glaciers commonly exhibit longitudinal ridges (e.g. Dufresne et al., 2019; McSaveney, 1978), the presence of landslides with longitudinal ridges has been used to infer the past presence of ice on Mars (De Blasio, 2011; Gourronc et al., 2014; Lucchitta, 1979, 1987), where hundreds of well-preserved long-runout landslides with longitudinal ridges are found. However, the presence of the same landforms and associated morphologies in regions where extensive glaciations did not occur, for instance, on the Moon (e.g. Boyce et al., 2020) and in the Atacama region on Earth (Mather et al., 2014), suggests that ice is not the only factor influencing the formation of long-runout landslides with longitudinal ridges.
On Earth, there are not as many well-preserved cases of long-runout landslides with associated longitudinal ridges as there are on Mars. Such difference might suggest that longitudinal ridges in long-runout landslides do not commonly form on our planet. However, more than 100 landslides characterised by longitudinal ridges are easily identifiable in Iceland (e.g. Decaulne et al., 2016; Mercier et al., 2013), making it an exceptional region on Earth for its high spatial density of such landforms. The unique record of long-runout landslides with longitudinal ridges in Iceland suggests that either conditions in Iceland, at the time of landslide formation, were optimal for the development of these landforms or longitudinal ridges are indeed common in long-runout landslides on Earth, but there is a preservation bias in Iceland. The study of long-runout landslides with longitudinal ridges in Iceland represents an opportunity to investigate the formation mechanisms of such hypermobile landslides and their possible link with past environmental conditions. A better understanding of the influence of climatic, geological, and environmental conditions in the development of hypermobile landslides will have implications for both terrestrial hazard assessment and reconstruction of extra-terrestrial palaeo-environments.
In this study, we conducted a detailed survey of landslides in Iceland to identify and compile the first Icelandic catalogue of long-runout landslides with longitudinal ridges. We compared the morphological parameters of Icelandic landslides with Martian landslides that are similar in length. We present detailed morphological observations of one Icelandic case study, the Dalvík landslide, and we compare them with morphological observations of analogue Martian landslide deposits. We show that Icelandic long-runout landslides share similar morphometric values and diagnostic longitudinal ridges to those observed in Martian long-runout landslides. Therefore, we conclude that Icelandic long-runout landslides with associated longitudinal ridges can be used as good morphological and morphometric analogues of similar-sized Martian landforms, as in the case for other elements of the Icelandic landscape and its associated mass-wasting processes (e.g. Conway et al., 2015, 2019; de Haas et al., 2015; Hartmann et al., 2003; Morino et al., 2019, 2023).
2.1 Icelandic long-runout landslides
In order to identify and map long-runout landslides with longitudinal ridges in Iceland, we used available high-resolution satellite/aerial imagery from Google Earth and the Icelandic Map website (https://www.map.is, last access: 31 January 2023). In ArcGIS, we used digital elevation models (DEMs) provided by ArcticDEM (Porter et al., 2018; 2 m px−1 resolution) and their hillshade models to extrapolate landslide morphometric data (elevation drop and horizontal length; H and L, respectively, in Table S1 in the Supplement). In combination with the image data, the hillshade was used to identify the locations of the extreme ends of the headscarp and toe of the deposit so their elevation values could be extracted from the DEM.
We acquired drone imagery of one Icelandic landslide near the town of Dalvík (I-landslide ID 47 in Table S1). We used 10 ground control points (GCPs) in order to correctly georeference the drone images (Table S2) acquired by differential GPS and marked by 0.8×0.8 m square orange targets easily visible in the images. We obtained the latitude and longitude coordinates (WGS84) and the height above the ellipsoid of 10 locations, of which 8 were on the landslide deposit and 2 were on the slope adjacent the distal frontal edge of the deposit (Fig. 4), using a Leica dGPS GS09. We occupied the points for >5 min and post-processed the data using the permanent GPS station in Akureyri (AKUR run by LMI Landmælingar Íslands – National Land Survey of Iceland) 38 km away from the site. The resulting positions had errors much smaller than the error produced by inherent uncertainty in identifying the control points in the drone images (the worst position had a standard deviation of 2.4 cm in vertical position, yet this was typically 3 mm). We calculated the elevation above the geoid (i.e. elevation above sea level) using an online geoid calculator (GeoidEval). We built a digital elevation model (4.65 cm px−1 resolution) and an orthoimage (2.38 cm px−1 resolution) of the Dalvík landslide using Agisoft Metashape (dataset provided in Magnarini et al., 2023).
2.2 Martian long-runout landslides
We made use of the landslide inventory provided by Crosta et al. (2018) to select Martian landslides with longitudinal ridges that have a comparable length to Icelandic long-runout landslides with longitudinal ridges. We selected Martian landslides that are shorter than 5 km long (Table S3), which is double the length of the longest Icelandic landslide considered in this study (I-landslide ID 103; L=2629 m). We added additional landslides with longitudinal ridges that are not found in the Crosta et al. (2018) inventory, which were found using high-resolution satellite imagery acquired by the ConTeXt (CTX) camera (6 m px−1 resolution; Malin et al., 2007) and, where available, the High-Resolution Imaging Science Experiment (HiRISE) camera (nominal resolution 25 cm px−1; McEwen et al., 2007) on board the NASA Mars Reconnaissance Orbiter (MRO). In total, we selected 112 landslides. As Martian landslides can be up to 70 km long (Lucchitta, 1979), those selected for this work will be also referred to as “small-scale”, as done in the literature (Guimpier et al., 2021).
In ArcGIS, we calculated the elevation drop (H) and measured the horizontal length (L) using the HRSC MOLA Blended global DEM at 200 m px−1 resolution (Fergason et al., 2018). CTX images were used to identify the top of the headscarp and toe of the deposit so that their elevation values could be extracted from the DEM. We generated digital elevation models and orthoimages of four landslides (M-landslide ID 31; M-landslide ID 42; M-landslide ID 46; M-landslide ID 47) using CTX (Malin, 2007; Malin et al., 2007) image pairs (Table S4). CTX-derived DEMs were generated using the United States Geological Survey (USGS) Integrated Software for Imagers and Spectrometers (ISIS) software and commercial image analysis software SOCET SET (Kirk et al., 2008). DEMs were vertically and horizontally controlled to Mars Orbital Laser Altimeter topographic data (Smith et al., 1999; Zuber et al., 1992). The resolution of CTX DEMs and orthoimages is 20 and 6 m px−1, respectively. We estimated the vertical precision of the CTX-derived DEMs using the established method of Okubo (2010) (Table S4).
Paraglacial adjustment, that is the readjustment of glaciated landscapes to non-glacial conditions following deglaciation, is considered a critical cause of slope instability in high-latitude environments (e.g. Ballantyne and Stone, 2004; Wyrwoll, 1977). According to the “exhaustion model” (Ballantyne, 2002; Cruden and Hu, 1993), during the paraglacial adjustment, the occurrence of mass-wasting decreases exponentially over time after deglaciation. In Iceland, the occurrence of large landslides is attributed to ice retreat following the Last Glacial Maximum (LGM), either by the effect of glacial debuttressing or glacial rebound (e.g. Ballantyne, 2002; Coquin et al., 2015; Cossart et al., 2014; Fernández-Fernández et al., 2022; Saemundsson et al., 2022; Vick et al., 2021) or by pressurised water derived from glacial melting (Whalley et al., 1983). The ages available for large landslides in Iceland fit the paraglacial exhaustion model. Most of the dated landslides occurred in the first half of the Holocene (Coquin et al., 2015; Cossart et al., 2014; Decaulne et al., 2016; Mercier et al., 2013, 2017), consistent with the Icelandic landslide theory of Jónsson (1957), according to which landslide activity initiated shortly after the retreat of the ice sheet at the end of the LGM. However, out of more than 100 landslides, only a few have been dated (Decaulne et al., 2016; Mercier et al., 2013, 2017), and patterns of landslide occurrence following deglaciation are still debated (e.g. Cossart et al., 2014). Therefore, more data are needed to better constrain the relationship between the occurrence of large landslides and the effects of deglaciation.
Iceland has a unique high spatial density of well-preserved hypermobile large landslides with longitudinal ridges (Figs. 2 and 3). This type of landslide is commonly found in the Tröllaskagi peninsula, in northern–central Iceland (Fig. 4). This region is constituted of Tertiary basalt lava flows alternated with weathered red palaeosols (Johannesson and Saemundsson, 1989; Saemundsson, 1980; Thordarson and Höskuldsson, 2002), the latter considered weak layers within which slope failure planes could potentially develop (Jónsson, 1957; Mercier et al., 2013). Three landslides with longitudinal ridges have been dated (here we report the ages as provided in the literature; for further details about the dating techniques used, age–depth models, and uncertainties, the reader should refer to the cited literature): the Vatn landslide (I-landslide ID 69 in this work) is thought to have occurred between 11 400 and 10 790 cal yr BP (Decaulne et al., 2016); the Höfðahólar landslide (I-landslide ID 118 in this work) is thought to have occurred between 10 200 and 7975 cal yr BP (Mercier et al., 2013); and the Flókadalur landslide (I-landslide ID 57 in this work) is thought to have occurred between 15 577±1455–11 002±2778 cal yr BP (Mercier et al., 2017).
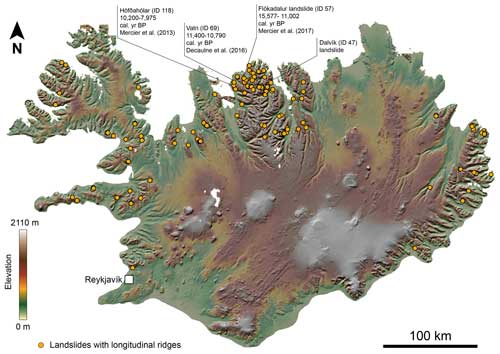
Figure 2Distribution of long-runout landslides with longitudinal ridges in Iceland. The yellow dots represent locations of 129 long-runout landslides with longitudinal ridges, for which geographic coordinates are given in Table S1. The map shows the location of the three landslides with longitudinal ridges that have been dated by previous work and the location of the Dalvík landslide investigated in this work. The base map is the hillshaded and colour-keyed 500 m px−1 resolution ArcticDEM (Porter et al., 2018).
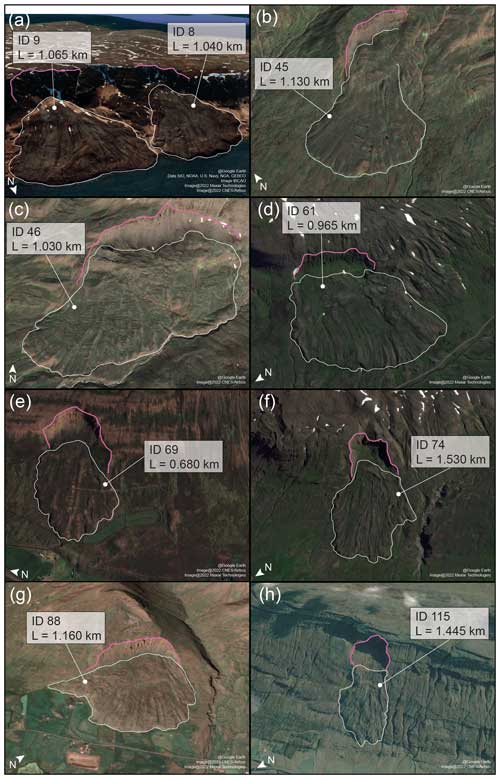
Figure 3Examples of long-runout landslides with ridges in Iceland. The white lines identify the landslide deposits and the pink lines identify the headscarps. For each landslide, ID and length (L) are provided. For the full list of the landslides catalogued in this work, see Table S1.
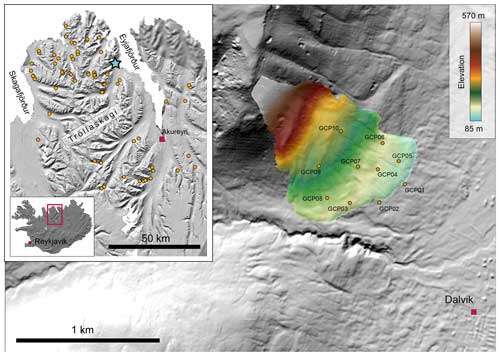
Figure 4The Dalvík landslide in the Tröllaskagi peninsula. The landslide is located near the town of Dalvík. The landslide is ID 47 in the Icelandic catalogue of long-runout landslides with longitudinal ridges that we compiled in this work. The large panel shows in colour the DEM generated from drone imagery overlain on a hillshaded render of the ArcticDEM, and the points indicate the location of 10 GCPs used to georeference the DEM. The inset shows the location of the Dalvík landslide in Tröllaskagi peninsula (blue star), along with the other landslides in this work (orange dots), showing that it is the Icelandic region with the highest landslide density. The location of the Tröllaskagi peninsula within Iceland is shown in the bottom-left corner of the inset with a red box. The inset uses the hillshaded ArcticDEM as a basemap (Porter et al., 2018).
3.1 The Dalvík landslide
In this study, we investigated a landslide in the field located 1 km northwest of the town of Dalvík in the Tröllaskagi peninsula (Fig. 4). The Dalvík landslide (I-landslide ID 47) is a good example of a well-preserved long-runout landslide with longitudinal ridges in Iceland. The landslide has a horizontal runout (L) of 1.210 km and a vertical drop (H) of 0.480 km (Fig. 1), resulting in a ratio of 0.396.
Similar to other long-runout landslides, the edges of the deposit are steep (on average about 30° slope) and about 15–25 m high (Fig. 5d). The bedrock in the headscarp exposure shows typical alternation of Tertiary lava layers and red palaeosols (Fig. 5a and b). The landslide deposit sits on a sedimentary cover that has an undulating surface appearance (Fig. 5c) and could be due to previous mass movements or glacial deposits. The zone of depletion is characterised by a Toreva block (about 200 m long, 450 m wide, and 80 m high), while the zone of accumulation is composed of a debris apron about 750 m long. Boulders decimetres to metres in size are found on the surface of the deposit. However, size sorting of boulders from proximal to distal area of the landslide seems not to occur.
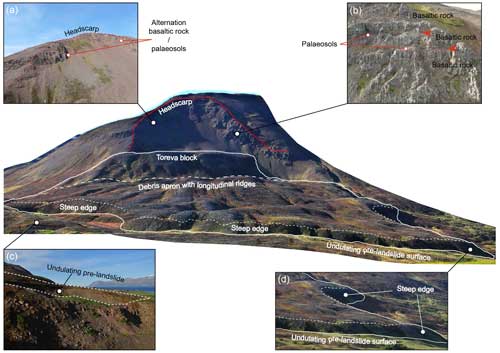
Figure 5The Dalvík landslide. The image in the centre shows a perspective view of the drone photomosaic of the Dalvík landslide. Annotations highlight the main morphological features and structures. Panels (a) and (b) are photos acquired by a drone, showing details of the rock formations that characterise the headscarp. Panel (c) shows an outcrop of the unsorted sediments that form the undulating pre-landslide surface over which the Dalvík landslide is found. Panel (d) shows a closer view of the 15–25 m high steep termination of the landslide deposit.
The debris apron is characterised by longitudinal ridges which can be identified from both aerial/satellite images and ground photos. The longitudinal ridges occur across the entire length of the debris apron (Fig. 6). At some locations, longitudinal ridges bifurcate (e.g. locations marked with a star in Fig. 6; red arrowheads in Fig. S2a and b), forming two ridges commonly narrower than the parent ridge. Consequently, the number of longitudinal ridges increases from the proximal to the distal area of the apron. Transverse ridges appear only on the SW side of the deposit.
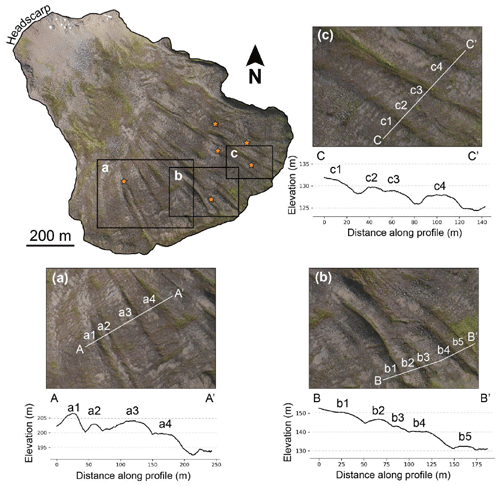
Figure 6Morphology of longitudinal ridges in the Dalvík landslide. The image shows the high-resolution orthoimage of the Dalvík landslide generated from drone-acquired photos. The orthoimage provides complete view of the extent of longitudinal ridges over the landslide deposit. Panels (a)–(c) show close-up views of longitudinal ridges, whose morphology is shown by topographic profiles extracted from the profiles (A–A′, B–B′, and C–C′, respectively), and the annotations a1, a2, etc., indicate corresponding ridges in the images and profiles. The stars show locations where longitudinal ridges bifurcate, resulting in the development of two ridges from a parent ridge.
In addition to longitudinal ridges, the Dalvík landslide exhibits other linear structures superposed on, and broadly transverse to, the longitudinal ridges (Fig. 7). These linear structures appear to have en echelon configuration. The en echelon structures appear to be oblique to the longitudinal direction of the ridges. Towards the frontal edge of the deposit, the orientation of these linear structures changes and gradually becomes perpendicular to the extension of the ridges, which corresponds to the direction of movement of the landslide.
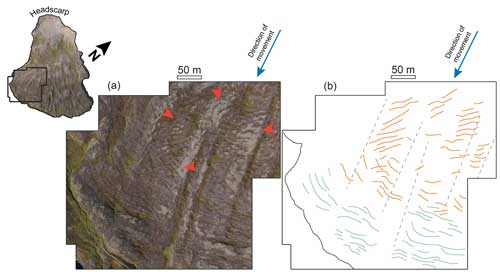
Figure 7En echelon structures found superposing longitudinal ridges of the Dalvík landslide. (a) Drone-acquired image mosaic of the SW terminal part of the Dalvík landslide. Panel (b) shows digitised en echelon features observed on the longitudinal ridges in panel (a). Orange lines represent the linear structures that are oblique to the direction of the ridges, which are shown with dashed black lines. Green lines represent the linear structures that are transverse to the direction of the ridges. This map shows how the transition from oblique to transverse occurs towards the terminal part of the deposit. Blue arrows show the direction of movement of the landslide; red arrowheads show locations where en echelon linear structures are found superposing longitudinal ridges.
3.2 Catalogue of Icelandic long-runout landslides with longitudinal ridges
We compiled the first catalogue of Icelandic long-runout landslides with longitudinal ridges, which comprises of 129 of these landforms (Table S1). We included only landslides that have distinct longitudinal ridges, and we omit those landslides for which the presence of longitudinal ridges was uncertain. Ridges were identified by their horizontal continuity in the downslope direction, and their presence is evaluated using a top-view observation of the landslide deposit.
The horizontal length of the Icelandic landslides spans from 0.287 km (I-landslide ID 127) to 2.629 km (I-landslide ID 103). The median and mean values of length are 1037 and 1150 m, respectively. The median and mean values of elevation drops are 317 and 337 m, respectively. The ratio of this set of Icelandic long-runout landslides ranges from 0.149 (I-landslide ID 18) to 0.58 (I-landslide ID 42). The statistics of the morphometry of Icelandic landslides are summarised in Figs. 12 and 13.
Some of the landslides have their headscarps at the top of cliffs and mountains (e.g. I-landslide ID 8, ID 9 in Fig. 3), whereas other cases develop from the mid-/lower part of slopes (e.g. I-landslide ID 74 and ID 88 in Fig. 3). All the landslide deposits are characterised by steep edges, typically about 20 m high. About 25 % of the landslides have a Toreva block. Another characteristic found in some landslides is the presence of lateral levees that develop continuously from the depletion zone. In about the 80 % of the cases, the deposit does not entirely reach the slope break at the base of the slope. The landslides often rest on the inclined surface that connects the slope to the bottom of the valley (e.g. I-landslide IDs 45, 69, and 74, ID 115 in Fig. 3, and the Dalvík landslide). Such connecting surfaces have slopes of about 8–15°. They correspond to sedimentary units, whose origin cannot be established from remote sensing only (in Fig. 5, such connecting surface is labelled “undulating pre-landslide surface”). A river gorge near the Dalvík landslide exposes outcrops showing the sedimentary origin of the inclined surface over which the landslide deposited (Fig. 5c). In the case of absence of the connecting inclined surface, landslides extend beyond the slope break at the base of the slope (e.g. I-landslide ID 8 and ID 9 in Fig. 3).
Geomorphological observations have been used to provide evidence of paraglacial periods on Mars (e.g. Gourronc et al., 2014; Jawin et al., 2018; Jawin and Head, 2021; Mège and Bourgeois, 2011) that immediately postdate glacial retreat. These are characterised by an assemblage of specific landforms that develop in response to ice removal, glacial unloading, and the exposure of steep slopes and large sediment stores (e.g. Ballantyne, 2002). In Valles Marineris, structures diagnostic of deep-seated gravitational slope deformation have been described (Gourronc et al., 2014; Mège and Bourgeois, 2011), and their development has been interpreted as the consequence of slope debuttressing and decohesion following the retreat of glaciers (Makowska et al., 2016; Mège and Bourgeois, 2011).
Mège and Bourgeois (2011) suggest that the large long-runout landslides in Valles Marineris are the result of an advanced stage of paraglacial, deep-seated gravitational slope deformation that led to large-scale catastrophic slope failures. Moreover, Mège and Bourgeois (2011) and Gourronc et al. (2014) conclude that the long-runout landslides in Valles Marineris originated as supraglacial landslides based on two observations: first, the emplacement on ice could explain the excessive runout of the landslides (e.g. De Blasio, 2011, 2014; Dufresne et al., 2019; Dufresne and Davies, 2009; Lucas et al., 2011, 2014; Shreve, 1966); and second, the longitudinal ridges that characterise their deposits are considered a morphological signature of landslides emplaced on glaciers (De Blasio, 2011; Dufresne and Davies, 2009). However, Magnarini et al. (2019) argue that the presence of an icy surface is not necessary to develop longitudinal ridges in long-runout landslides on Mars (as also supported by the existence of a long-runout landslide with longitudinal ridges on the Moon; e.g. Boyce et al., 2020), which instead could develop from a mechanical instability imparted by high-velocity granular flow mechanisms.
Martian long-runout landslides with longitudinal ridges
In this work, we compiled a catalogue of Martian long-runout landslides shorter than 5 km long that exhibit longitudinal ridges (Table S3; Figs. 8–10) and are thus comparable to the length of Icelandic long-runout landslides with longitudinal ridges. We only included long-runout landslides that have distinct longitudinal ridges, and we did not consider landslides where the presence of longitudinal ridges is uncertain. Moreover, we have excluded deposits with longitudinal ridges that do not have matching headscarps. The catalogue that we compiled comprises of 112 of these landforms, which represent the 3 % of the latest Martian landslide database (3118 entries) provided by Crosta et al. (2018).
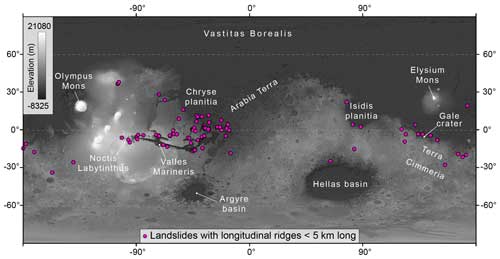
Figure 8Distribution of long-runout landslides (less than 5 km long) with longitudinal ridges on Mars. The pink dots represent the locations of 112 long-runout landslides with longitudinal ridges, with geographic coordinates given in Table S3. The base map is a greyscale rendering of the HRSC MOLA-blended global digital elevation model (200 m px−1 resolution). Some examples of the landslide deposits included in this catalogue are shown in Fig. 10.
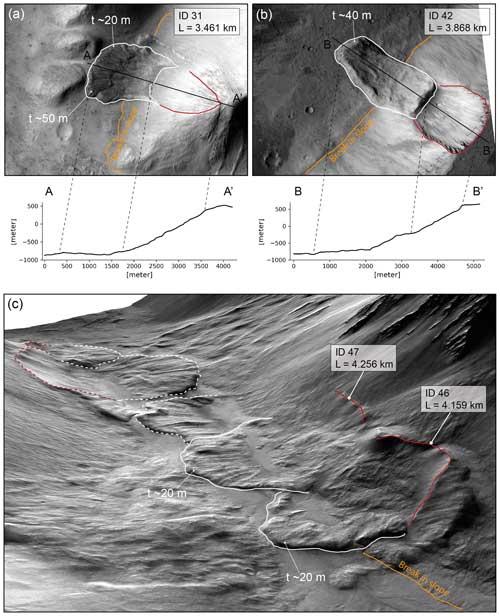
Figure 9Martian long-runout landslides with longitudinal ridges. Panels (a) and (b) show top views of landslide ID 31 (CTX image D16_033578_1752_XN_04S084W) and landslide ID 42 (CTX image D21_035252_2083_XN_28N072W) and their longitudinal topographic profiles; in both panels, north is up. Panel (c) is an oblique view of a cluster of landslides with longitudinal ridges (J16_050944_1665_XN_13S065W). Red lines show headscarps. White lines show landslide deposits (dashed red and white lines are for landslides not included in the catalogue because they are either too long (>5 km) or missing the headscarp). Orange lines show the base of the slope. In each panel, the landslide ID, lengths, and thickness (t) values at the frontal edge are provided.
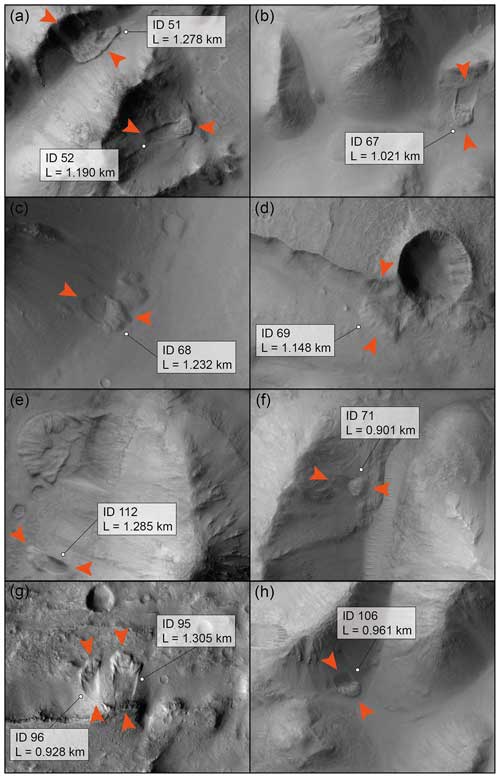
Figure 10Examples of Martian long-runout landslides shorter than 5 km long that exhibit longitudinal ridges. The orange arrowheads show the approximation location of the headscarp and of the toe of the landslides. For each landslide, ID and length are provided. For the full list of the landslides catalogued in this work, see Table S3. In all panels, north is up. (a) CTX image P16_007244_1796_XN_00S045W; (b) CTX image P19_008417_1807_XN_00N036W; (c) CTX image P05_002800_1814_XI_01N035W; (d) CTX image P02_002022_1801_XN_00N035W; (e) CTX image P16_007349_1831_XN_03N032W; (f) CTX image B19_017001_1789_XN_01S032W; (g) CTX image P04_002642_1717_XI_08S041W; and (h) CTX image B19_017001_1789_XN_01S032W. Image credit: NASA/JPL/MSSS.
The horizontal length of the Martian landslides included in the catalogue spans from 0.508 km (M-landslide ID 3) to 4.902 km (M-landslide ID 43). The median and mean values of length are 1930 and 1803 m, respectively. The median and mean values of elevation drops are 527 and 536 m, respectively. The ratio of this set of Martian long-runout landslides ranges from 0.076 (M-landslide ID 14) to 0.468 (M-landslide ID 12). The statistics of the morphometry of small Martian landslides is summarised in Figs. 12 and 13.
Small-scale Martian long-runout landslides with longitudinal ridges are found between 38° N and 34° S. The largest concentration is found in the chaotic terrains east of Valles Marineris and in the channels and valleys that flow into Chryse Planitia. Other major clusters are found in Noctis Labyrinthus and north of Terra Cimmeria. Some of the landslides have their headscarps at the top of cliffs and mountains (e.g. in Figs. 9a, b and 10a, g), whereas other cases develop from the lower part of slopes (e.g. in Figs. 9c and 10b, c, e, f, h). All the landslide deposits are characterised by steep edges, typically about 20–50 m high. Only about 20 % of these landslides have a Toreva block. Another typical characteristic is the presence of lateral levees that develop continuously from the depletion zone (e.g. Fig. 11a). The debris aprons rest on sub-horizontal surfaces, which are either valley or crater floors. In only five cases (M-landslide ID 67, 71, 79, 102,and 112) does the deposit not entirely reach the slope break at the base of the slope (e.g. in Fig. 10b, e and f).
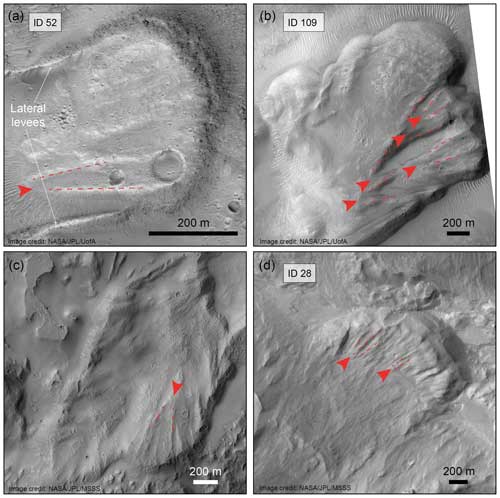
Figure 11Behaviour of longitudinal ridges in small-scale (<5 km long) Martian long-runout landslides. The red arrowheads show the locations where longitudinal ridges bifurcate, resulting in the development of two ridges from a parent ridge. Dashed red lines show the orientation of longitudinal ridges. Panels (a), (b), and (d) show examples of landslides included in this work and whose number ID is provided in the figure (HiRISE images: ESP_040790_1805, ESP_070830_1795 and ESP_026497_1755, respectively. Image credit: NASA/JPL/UofA). Panel (c) shows an example of bifurcating longitudinal ridges in a landslide not included in the list presented in this work, as its length is slightly more than 5 km (CTX image: P01_001337_1757_XN_04S063W. Image credit: NASA/JPL/MSSS). In all panels, north is up.
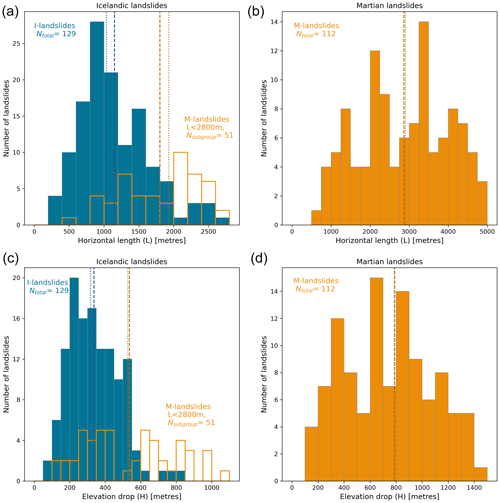
Figure 12Morphometric results. The data in a sea blue represent the Icelandic landslide population. The data in gold represent the Martian landslides shorter than 5 km long. Top panels show the distribution of landslide lengths. The lower panels show the distribution of landslide elevation drops. In the panels showing Icelandic landslides, the data relative to the subgroup of the Martian landslides with maximum length shorter than or equal to the maximum length of the Icelandic landslides are also plotted. Dashed lines represent the mean and dotted lines represent the median.
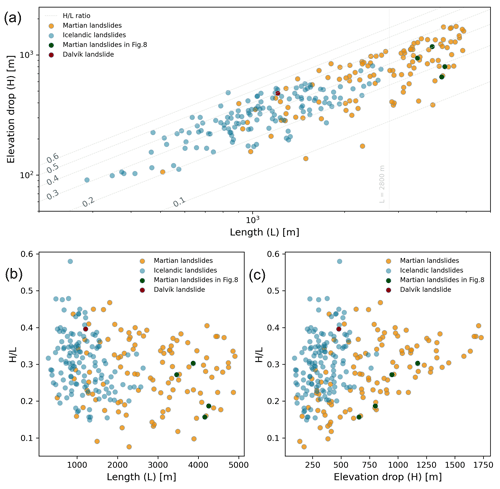
Figure 13 ratio. In panel (a) the elevation drop (H) is plotted against the horizontal length (L) to visually represent the ratios that characterise the landslides compiled in this study. The dashed grey lines represent constant ratios, whose values are given at the left-hand side of each line. The vertical light grey line shows the value of L=2800 m, which corresponds to the maximum length of the Martian landslide subgroup. Axes are given in logarithmic scale. Panels (b) and (c) show the ratio plotted against the horizontal length (L) and the elevation drop (H), respectively.
Longitudinal ridges are visible in both CTX and HiRISE images across the entire length of the debris aprons. Similar to what we observed at the Dalvík landslide, longitudinal ridges split, generating two ridges from a parent ridge (Fig. 11). Unfortunately, the resolution of the CTX-derived DEMs (20 m px−1) is not able to resolve the topography of longitudinal ridges; and no HiRISE image pairs are available to generate higher-resolution DEMs of the landslides discussed in this work. Therefore, a topographic comparison between longitudinal ridges of the Martian and the Dalvík landslide could not be conducted.
In this work, we used the selected small-scale Martian long-runout landslides to conduct morphometric and morphological comparisons with Icelandic landslides with longitudinal ridges because they share not only morphometric similarities but also morphological structures. In addition to longitudinal ridges, they are all characterised by steep terminal edges and can develop lateral levees or Toreva blocks. Additionally, the headscarps of both the Icelandic and Martian landslide populations are found over a range of elevations along the slopes (i.e. they are not limited to the top of cliffs but also occur at mid- to lower sections of slopes; see Fig. 9c, landslide ID 46, and Fig. 10a, c, e, f and h, landslide IDs 52, 68, 112, 71, and 106, respectively). Only five Martian landslides do not entirely reach the slope break at the base of the slope, whereas more than 80 % of the Icelandic landslide deposits rest on the inclined surface that connects the slope to the bottom of the valley.
In the following sections, we discuss the results from the morphometric and morphological comparisons and draw considerations on their implications about the formation of landslides with longitudinal ridges on Mars and in Iceland.
5.1 Morphometric comparison
The gigantic, long-runout landslides on Mars have attracted much interest, giving rise to a commonly accepted belief that Martian landslides are an order of magnitude larger than terrestrial landslides (e.g. Brunetti et al., 2014; Legros, 2002; Lucchitta, 1978, 1979; McEwen, 1989). However, the availability of higher-resolution orbital imagery has shown the existence of Martian long-runout landslides that are smaller and similar in size to terrestrial long-runout landslides (Crosta et al., 2018; Guimpier et al., 2021, 2022).
The mobility of long-runout landslides, expressed as ratio, increases with increasing volume (Heim, 1932; McEwen, 1989; Legros, 2002). When the largest landslides are taken into account, terrestrial and Martian long-runout landslides seem to show distinctive trends, suggesting that Martian long-runout landslides are less mobile than terrestrial long-runout landslides; that is, for long-runout landslides with equal volume, the terrestrial ratio is lower than the Martian one. However, such a trend becomes less obvious when larger datasets that include smaller landslides are considered (Crosta et al., 2018), and the large scatter of the data points in the vs. L plot suggests that other parameters affect the mobility of long-runout landslides.
When volume values are not available, length (L) is used as a proxy for the volume of landslides (Legros, 2002; Singer et al., 2012; Lucas et al., 2014; Johnson and Campbell, 2017; Schmidt et al., 2017; Beddingfield et al., 2020; Johnson and Sori, 2020). In this work, we used L as a proxy for landslide volume (Fig. 13b).
Figure 13a is a visual representation of the ratio that characterises the populations of the landslides that we have analysed in this study. The plot shows that the ratio range that characterises the two landslide populations overlaps and that there is no distinct trend in the ratio of the Icelandic and small-scale Martian landslides. When instead plotted against elevation drop (Fig. 13c), the ratio shows a correlation that is increasing with increasing fall height. This trend was shown to also exist for large landslides on Earth, Mars, and Iapetus by Johnson and Campbell (2017) and confirmed by their numerical simulations. The plot in Fig. 13a also seems to show that there are two distinct lower boundaries for the Martian and Icelandic landslides.
In order to isolate and test the effects of the length and elevation drop on the mobility, we locked the landslide populations to equivalent maximum length. Therefore, we restricted the selected Martian landslides to a smaller subset (Nsubgroup=51), whose maximum length matches that of the Icelandic landslides (L<2800 m). It is interesting that these two populations with equivalent L (that is, with assumed equivalent volume) show similar mobility (Icelandic average ratio = 0.303; Martian average ratio = 0.292), thus supporting the absence of distinctive trends of mobility in small-scale terrestrial and Martian long-runout landslides. Additionally, the Martian landslide subgroup shows cases that are characterised by higher-elevation drops (Icelandic max elevation drop = 810 m; Martian max elevation drop = 1071 m). As explained in Melosh (2011), the lower surface gravity of Mars means that for the same material strength Mars can support proportionally higher topography than Earth. These results are again in agreement with Johnson and Campbell (2017).
5.2 Flow structures and behaviour of longitudinal ridges
Lateral spreading of landslide deposits has been considered an important factor controlling the behaviour of longitudinal ridges and the thickness of a landslide deposit (Magnarini et al., 2021b). Unconfined long-runout landslides tend to spread laterally, causing the thinning of the deposit with distance and hence divergence of the longitudinal ridges. However, the existence of a scaling relationship between the thickness of the landslide and the spacing between the ridges (i.e. the distance between ridges ranges between 2 and 3 times the thickness of the deposit; Magnarini et al., 2019, 2021a, b) implies that as the landslide thins while laterally spreading, and then the distance between the ridges has to decrease accordingly. Therefore, the scaling relationship is maintained by the splitting of parent ridges and also by the appearance of new smaller ridges between spreading longitudinal ridges (Magnarini et al., 2019).
We observed longitudinal ridges bifurcating, thus splitting and developing two ridges from the parent ridge, in both the Dalvík landslide (Figs. 6 and S2a, b) and small-scale Martian landslides (Fig. 11). The resulting new ridges are less wide. This process leads to an increase in the number of longitudinal ridges with distance. Similar behaviour has been described for the 63 km long Martian Coprates Labes landslide, in Valles Marineris, by Magnarini et al. (2019) (Fig. S2c and d). Therefore, the occurrence of ridge splitting seems to be scale-independent. However, in the Dalvík landslide, we do not observe the appearance of new, smaller ridges between two existing ridges, which is observed in the small-scale Martian landslide ID 109 (Fig. 11b). Similar ridge behaviour is observed in two other Martian landslides that are not included in the current selection, namely the landslide shown in Fig. 11c and the Coprates Labes landslide (Magnarini et al., 2019).
The fact that the Dalvík landslide shows only the splitting of parent longitudinal ridges into two smaller ridges and does not show the appearance of new ridges between two existing ridges may be due to limited lateral spreading at Dalvík compared to the Martian examples. Limited lateral spreading in turn limits the thickness reduction in the deposit and limits the space for new ridges to develop. Lateral spreading may be limited in Dalvík by the basal surface over which the landslide moved and thus not providing sufficiently low friction, the rheology of the landslide prevented it from spreading further laterally, or the landslide was not large enough and thus did not have enough kinetic energy to expand further. The latter proposed explanation may have support in the fact that the landslides in which we report the appearance of new ridges (in addition to ridge splitting) are all much larger than the Dalvík landslide.
Similar to the Dalvík landslide, linear en echelon structures are seen in an 8 km long Martian landslide (e.g. Fig. 14a and b) and have also been described in the 63 km long Coprates Labes landslide by Magnarini et al. (2019) (Fig. 14c and d). Similar linear structures superposed on longitudinal ridges were also described in the Sherman Glacier landslide deposit, Alaska, as “transverse fissures” by Shreve (1966) and McSaveney (1978); in the Lamplugh landslide, Alaska, by Dufresne et al. (2019); and in the Iymek landslide, China, by Shi et al. (2022). Such linear structures have been interpreted as kinematic indicators, as they indicate the differential velocity of adjacent sections of the landslides during emplacement (e.g. Dufresne et al., 2019; Magnarini et al., 2019; McSaveney, 1978; Shreve, 1966).
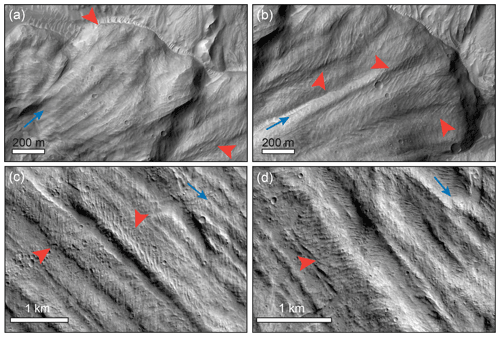
Figure 14En echelon structures found superposing longitudinal ridges in Martian long-runout landslides. Panels (a) and (b) show examples of linear structures superposed on longitudinal ridges in an 8 km long landslide on Mars (HiRISE image: ESP_016778_1750). Panels (c) and (d) show examples from the 63 km long Coprates Labes landslide deposit on Mars (CTX image: P20_008906_1685_XN_11S067W). In all panels, blue arrows show the direction of movement of the landslides; red arrowheads show locations where en echelon linear structures are found superposing longitudinal ridges (note that these are examples, and the structures are found ubiquitously on the deposits).
In the Dalvík landslide, towards the frontal edge of the deposit, these linear structures change orientation and gradually become perpendicular to the direction of the ridges (Fig. 7), which corresponds to the direction of movement of the landslide. The observed change in the orientation of the en echelon structures may represent the expression of changing velocity during emplacement; the oblique configuration shows that different parts of the deposit were characterised by different velocities, and the transition to transverse configuration, i.e. perpendicular to the landslide movement direction, shows that different parts of the landslide progressively attained similar velocity. Given that the oblique–transverse transition occurs towards the terminus of the deposit, it may represent evidence of slowing down of the landslide. However, such a transition does not seem to take place towards the terminal part of the Martian landslide in Fig. 14a and b; therefore, either a different interpretation is required or the Martian landslide came to a halt in a different manner than the Dalvík landslide.
5.3 Implications and future directions
In this work, we showed that small-scale Icelandic and Martian long-runout landslides share similar mobility and morphological characteristics. It is reasonable to consider that the high mobility that these landslides have in common and their morphological similarities are a result of a similar mechanism of emplacement. However, the geomorphological issue of “equifinality” (i.e. similar landforms originating from different processes) is a major challenge in the interpretation of Martian landforms, as in situ data are limited to robotic exploration at few localised sites, and must be taken into consideration when linking morphology to processes (e.g. Balme et al., 2011). It follows also that interpretations based on morphological analysis may be biased due to morphological similarity (Johnson and Campbell, 2017; Magnarini et al., 2019; Johnson and Sori, 2020). With this in mind, we can, nevertheless, attempt to draw considerations about the implications of such similarities in terms of the emplacement mechanism, emplacement environment, and different gravity.
The effect of gravity on the formation of long-runout landslides on different planetary bodies is highly debated, and the matter is not settled yet. In this work, we have attempted to remove biases that populations of landslides with non-negligible size differences could carry with them. Our results do not suggest that gravity affects the mobility, as we do not see distinct trends between Martian and Icelandic landslides in our plots. Simulating terrestrial and Martian landslides, Johnson and Campbell (2017) reached similar conclusions that the lower surface gravity does not lead to significantly reduced mobility.
To our knowledge, no other region on Earth shows the same high spatial density of long-runout landslides with longitudinal ridges as Iceland does. Such unicity suggests that since the LGM (which broadly corresponds to the age of Icelandic landslides; Decaulne et al., 2016; Mercier et al., 2013, 2017), no other terrestrial setting has had conditions favourable for their development, and also preservation, to such a scale. On Mars, small-scale long-runout landslides with longitudinal ridges are not characterised by a high spatial density distribution, as in Iceland, although they tend to occur in steep-slope settings provided by chasmata, outflow channels, and chaotic terrains. However, there is evidence that the Martian record of small-scale landslides could have been largely removed. In fact, we identified several deposits almost fully obliterated by sedimentary burial (Fig. S1a and b in the Supplement). One of the best examples is found in Coprates Chasma, Valles Marineris, on Mars, where we found evidence of landslides with longitudinal ridges that have been partially buried and subsequently exhumed (Fig. S1c). The incomplete record of these landforms hampers our reconstruction of regions where conditions were favourable for their formation. In addition to the matter of where, the incomplete record prevents us from establishing when such conditions were optimal. Based on the existing age estimation of the largest long-runout landslides on Mars, long-runout landslides in Valles Marineris span from 3.5 Gya to 50 Ma (Quantin et al., 2004; Hager and Schedl, 2017); near Hydraotes Chaos, they span from 835 to 252 Ma (Molaro et al., 2024); and younger, small-scale landslides (<2 km long) have been found to be younger than 20 Myr old (Guimpier et al., 2021). According to these results, it appears that long-runout landslides on Mars have been recurring throughout much of Martian history, suggesting that the formation mechanism of long-runout landslides and their longitudinal ridges is persistent or recurrent in time.
The age difference between Icelandic and Martian landslides simply reflects different landslide populations of which we have accessible records. Therefore, it is not possible to establish whether the lack of records of Martian landslides more recent than 20 Ma is evidence that the mechanism favourable to their formation may have become unavailable on Mars.
The availability of an extensive, well-preserved, geomorphological record of long-runout landslides with longitudinal ridges in Iceland represents a unique opportunity to study their formation mechanisms and the influence of the environment on their development (both the geological and climatic contexts) at the regional scale. Reconstructing the palaeoenvironmental conditions at the time of their emplacement will help to better constrain the relationship between the occurrence of landslides with longitudinal ridges and the potential presence of ice or ice-cemented material (permafrost) during their emplacement. Not only will this represent important advancement in understanding the formation of these catastrophic landslides on Earth, but it will also provide insights into possible past Martian scenarios. However, formation mechanisms of long-runout landslides with longitudinal ridges that solely rely on climatic and environmental conditions should be carefully considered, especially on Mars, as it is well established that the planet has undergone dramatic climatic changes throughout its geological history (e.g. Laskar et al., 2004; Head et al., 2003; Bibring et al., 2006; Kite and Conway, 2024).
We compile the first catalogue of long-runout landslides with longitudinal ridges in Iceland and an equivalent catalogue of small-scale (<5 km long) landslides with longitudinal ridges on Mars. We measured the length and drop height in both catalogues and found significant overlap between the populations. In addition to morphometric comparability, our morphological observations show that Icelandic long-runout landslides share similar structural behaviours with Martian analogue deposits, such as splitting of longitudinal ridges and development of en echelon features. Therefore, this supports our hypothesis that Icelandic long-runout landslides with longitudinal ridges represent good morphological analogues of Martian landforms. The large record of long-runout landslides with longitudinal ridges emplaced after the Last Glacial Maximum in Iceland offers a unique opportunity to study the possible relation between the development of these landforms and glacial/paraglacial conditions. Unlocking this information will both contribute to tackling the challenges that current glaciated regions on Earth will face due to ongoing global warming and to gaining insights into Martian palaeoclimatic and palaeoenvironmental conditions.
The standard data products for Mars used in the study are available from the NASA PDS (2024) (https://pds.nasa.gov/). The shapefiles and topographic products generated for this work are provided through the data repository Figshare (https://doi.org/10.6084/m9.figshare.22333036.v1, Magnarini et al., 2023).
The supplement related to this article is available online at: https://doi.org/10.5194/esurf-12-657-2024-supplement.
GM: conceptualisation, investigation, fieldwork, and writing (original draft). AC: investigation. CM: writing (reviewing and editing). MP: fieldwork and writing (reviewing and editing). CB: fieldwork and writing (reviewing and editing). AD: writing (reviewing and editing). SJC: resources, investigation, fieldwork, and writing (reviewing and editing).
At least one of the (co-)authors is a member of the editorial board of Earth Surface Dynamics. The peer-review process was guided by an independent editor, and the authors also have no other competing interests to declare.
Publisher's note: Copernicus Publications remains neutral with regard to jurisdictional claims made in the text, published maps, institutional affiliations, or any other geographical representation in this paper. While Copernicus Publications makes every effort to include appropriate place names, the final responsibility lies with the authors.
This article is part of the special issue “Planetary landscapes, landforms, and their analogues”. It is not associated with a conference.
We thank reviewers Daniel Ben-Yehoshua and Ed Rhodes for their constructive comments which helped improve the quality and clarity of the paper. We thank associate editor Frances Butcher for handling this paper and providing additional comments.
Giulia Magnarini gratefully acknowledges UKRI Science Technology Facilities Council (STFC) for funding.
Susan J. Conway has been funded by the French space agency CNES for her HiRISE work. Susan J. Conway, Meven Philippe, and Calvin Beck have been funded by the Agence Nationale de la Recherche in the framework of the project ANR-19-CE01-0010 PERMOLARDS.
We acknowledge the financial support from the Observatoire Sciences de l'Univers Nantes Atlantique (OSUNA).
This research has been supported by the UK Research and Innovation (grant no. ST/V000799/1) and the Agence Nationale de la Recherche (grant no. ANR-19-CE01-0010 PERMOLARDS).
This paper was edited by Frances E. G. Butcher and reviewed by Daniel Ben-Yehoshua and Ed Rhodes.
Ballantyne, C. K.: A general model of paraglacial landscape response, Holocene, 12, 371–376, https://doi.org/10.1191/0959683602hl553fa, 2002.
Ballantyne, C. K. and Stone, J. O.: The Beinn Alligin rock avalanche, NW Scotland: cosmogenic 10Be dating, interpretation and significance, Holocene, 14, 448–453, https://doi.org/10.1191/0959683604hl720rr, 2004.
Balme, M. R., Bargery, A. S., Gallagher, C. J., and Gupta, S.: Martian Geomorphology: Introduction, in: Martian Geomorphology, edited by: Balme, M. R., Bargery, A. S., Gallagher, C. J., and Gupta, S., Geol. Soc. Lond. Spec. Publ., 356, 1–3, https://doi.org/10.1144/SP356.1, 2011.
Beddingfield, C. B., Beyer, R. A., Singer, K. N., McKinnon, W. B., Runyon, K., Grundy, W., Stern, S. A., Bray, V., Dhingra, R., Moore, J. M., Ennico, K., Olkin, C. B., Schenk, P., Spencer, J. R., Weaver, H. A., and Young, L. A.: Landslides on Charon, Icarus, 335, 113383, https://doi.org/10.1016/j.icarus.2019.07.017, 2020.
Bibring, J. P., Langevi, Y., Mustard, J. F., et al.: Global Mineralogical and Aqueous Mars History Derived from OMEGA/Mars Express Data, Science, 312, 400–404, https://doi.org/10.1126/science.1122659, 2006.
Boyce, J. M., Mouginis-Mark, P., and Robinson, M.: The Tsiolkovskiy crater landslide, the moon: An LROC view, Icarus, 337, 113464, https://doi.org/10.1016/j.icarus.2019.113464, 2020.
Brunetti, M. T., Guzzetti, F., Cardinali, M., Fiorucci, F., Santangelo, M., Mancinelli, P., Komatsu, G., and Borselli, L.: Analysis of a new geomorphological inventory of landslides in Valles Marineris, Mars, Earth Planet. Sc. Lett., 405, 156–168, https://doi.org/10.1016/j.epsl.2014.08.025, 2014.
Conway, S. J., Balme, M. R., Kreslavsky, M. A., Murray, J. B., and Towner, M. C.: The comparison of topographic long profiles of gullies on Earth to gullies on Mars: A signal of water on Mars, Icarus, 253, 189–204, https://doi.org/10.1016/j.icarus.2015.03.009, 2015.
Conway, S. J., de Haas, T., and Harrison, T. N.: Martian gullies: a comprehensive review of observations, mechanisms and insights from Earth analogues, Geol. Soc. Lond. Spec. Publ., 467, 7–66, https://doi.org/10.1144/SP467.14, 2019.
Coquin, J., Mercier, D., Bourgeois, O., Cossart, E., and Decaulne, A.: Gravitational spreading of mountain ridges coeval with Late Weichselian deglaciation: impact on glacial landscapes in Tröllaskagi, northern Iceland, Quaternary Sci. Rev., 107, 197–213, https://doi.org/10.1016/j.quascirev.2014.10.023, 2015.
Cossart, E., Mercier, D., Decaulne, A., Feuillet, T., Jónsson, H. P., and Sæmundsson, Þ.: Impacts of post-glacial rebound on landslide spatial distribution at a regional scale in northern Iceland (Skagafjörður), Earth Surf. Proc. Land., 39, 336–350, https://doi.org/10.1002/esp.3450, 2014.
Crosta, G. B., Frattini, P., Valbuzzi, E., and De Blasio, F. V.: Introducing a New Inventory of Large Martian Landslides, Earth Space Sci., 5, 89–119, https://doi.org/10.1002/2017EA000324, 2018.
Cruden, D. M. and Hu, X. Q.: Exhaustion and steady state models for predicting landslide hazards in the Canadian Rocky Mountains, Geomorphology, 8, 279–285, https://doi.org/10.1016/0169-555X(93)90024-V, 1993.
Davies, T. R. H. and McSaveney, M. J.: Mobility of long-runout rock avalanches, in: Landslides: Types, Mechanisms and Modeling, edited by: Stead, D. and Clague, J. J., Cambridge University Press, Cambridge, 50–58, https://doi.org/10.1017/CBO9780511740367.006, 2012.
De Blasio, F. V.: Landslides in Valles Marineris (Mars): A possible role of basal lubrication by sub-surface ice, Planet. Space Sci., 59, 1384–1392, https://doi.org/10.1016/j.pss.2011.04.015, 2011.
De Blasio, F. V.: Friction and dynamics of rock avalanches travelling on glaciers, Geomorphology, 213, 88–98, https://doi.org/10.1016/j.geomorph.2014.01.001, 2014.
Decaulne, A., Cossart, E., Mercier, D., Feuillet, T., Coquin, J., and Jónsson, H. P.: An early Holocene age for the Vatn landslide (Skagafjörður, central northern Iceland): Insights into the role of postglacial landsliding on slope development, Holocene, 26, 1304–1318, https://doi.org/10.1177/0959683616638432, 2016.
Dufresne, A. and Davies, T. R.: Longitudinal ridges in mass movement deposits, Geomorphology, 105, 171–181, https://doi.org/10.1016/j.geomorph.2008.09.009, 2009.
Dufresne, A., Bösmeier, A., and Prager, C.: Sedimentology of rock avalanche deposits – Case study and review, Earth-Sci. Rev., 163, 234–259, https://doi.org/10.1016/j.earscirev.2016.10.002, 2016.
Dufresne, A., Wolken, G. J., Hibert, C., Bessette-Kirton, E. K., Coe, J. A., Geertsema, M., and Ekström, G.: The 2016 Lamplugh rock avalanche, Alaska: deposit structures and emplacement dynamics, Landslides, 16, 2301–2319, https://doi.org/10.1007/s10346-019-01225-4, 2019.
de Haas, T., Hauber, E., Conway, S. J., van Steijn, H., Johnsson, A., and Kleinhans, M. G.: Earth-like aqueous debris-flow activity on Mars at high orbital obliquity in the last million years, Nat. Commun., 6, 7543, https://doi.org/10.1038/ncomms8543, 2015.
Fergason, L. R., Hare, T. M., and Laura, J.: HRSC and MOLA Blended Digital Elevation Model at 200 m v2, Astrogeology PDS Annex, US Geological Survey, http://bit.ly/HRSC_MOLA_Blend_v0 (last access: 2 May 2024), 2018.
Fernández-Fernández, J. M., Etzelmüller, B., Morino, C., and Sæmundsson, Þ.: Iceland, in: Periglacial Landscapes of Europe, edited by: Oliva, M., Nývlt, D., and Fernández-Fernández, J. M., Springer International Publishing, Cham, 427–473, https://doi.org/10.1007/978-3-031-14895-8_15, 2022.
Forterre, Y. and Pouliquen, O.: Longitudinal Vortices in Granular Flows, Phys. Rev. Lett., 86, 5886–5889, https://doi.org/10.1103/PhysRevLett.86.5886, 2001.
Gourronc, M., Bourgeois, O., Mège, D., Pochat, S., Bultel, B., Massé, M., Le Deit, L., Le Mouélic, S., and Mercier, D.: One million cubic kilometers of fossil ice in Valles Marineris: Relicts of a 3.5 Gy old glacial landsystem along the Martian equator, Geomorphology, 204, 235–255, https://doi.org/10.1016/j.geomorph.2013.08.009, 2014.
Guimpier, A., Conway, S. J., Mangeney, A., Lucas, A., Mangold, N., Peruzzetto, M., Pajola, M., Lucchetti, A., Munaretto, G., Sæmundsson, T., Johnsson, A., Le Deit, L., Grindrod, P., Davis, J., Thomas, N., and Cremonese, G.: Dynamics of recent landslides (<20 My) on Mars: Insights from high-resolution topography on Earth and Mars and numerical modelling, Planet. Space Sci., 206, 105303, https://doi.org/10.1016/j.pss.2021.105303, 2021.
Guimpier, A., Conway, S. J., Pajola, M., Lucchetti, A., Simioni, E., Re, C., Noblet, A., Mangold, N., Thomas, N., and Cremonese, G.: Pre-landslide topographic reconstruction in Baetis Chaos, mars using a CaSSIS Digital Elevation Model, Planet. Space Sci., 218, 105505, https://doi.org/10.1016/j.pss.2022.105505, 2022.
Hager, A. and Schedl, A. D.: Classification and Ages of Landslides Within Valles Marineris, in: 48th Lunar and Planetary Science Conference, 20–24 March 2017, The Woodlands, Texas, Abstract 2076, 2017.
Harrison, K. P. and Grimm, R. E.: Rheological constraints on martian landslides, Icarus, 163, 347–362, https://doi.org/10.1016/S0019-1035(03)00045-9, 2003.
Hartmann, W. K., Thorsteinsson, T., and Sigurdsson, F.: Martian hillside gullies and icelandic analogs, Icarus, 162, 259–277, https://doi.org/10.1016/S0019-1035(02)00065-9, 2003.
Head, J., Mustard, J., Kreslavsky, M., Milliken, R. E., and Marchant, D. R.: Recent ice ages on Mars, Nature 426, 797–802, https://doi.org/10.1038/nature02114, 2003.
Heim, A.: Der Bergsturz und Menschenleben, Fretz und Wasmuth Verlag, Zurich, 218 pp., 1932.
Hsü, K. J.: Albert Heim: Observations on Landslides and Relevance to Modern Interpretations, in: Developments in Geotechnical Engineering, vol. 14, Elsevier, 71–93, https://doi.org/10.1016/B978-0-444-41507-3.50009-X, 1978.
Jawin, E. R. and Head, J. W.: Patterns of late Amazonian deglaciation from the distribution of martian paraglacial features, Icarus, 355, 114117, https://doi.org/10.1016/j.icarus.2020.114117, 2021.
Jawin, E. R., Head, J. W., and Marchant, D. R.: Transient post-glacial processes on Mars: Geomorphologic evidence for a paraglacial period, Icarus, 309, 187–206, https://doi.org/10.1016/j.icarus.2018.01.026, 2018.
Johannesson, H. and Saemundsson, K.: Geological map of Iceland, 1:500 000, Bedrock geology, Icelandic Institute of Natural History, 1989.
Johnson, B.: Blackhawk Landslide, California, U.S.A., in: Developments in Geotechnical Engineering, vol. 14, Elsevier, 481–504, https://doi.org/10.1016/B978-0-444-41507-3.50022-2, 1978.
Johnson, B. C. and Campbell, C. S.: Drop Height and Volume Control the Mobility of Long-Runout Landslides on the Earth and Mars, Geophys. Res. Lett., 44, 12091–12097, https://doi.org/10.1002/2017GL076113, 2017.
Johnson, B. C. and Sori, M. M.: Landslide Morphology and Mobility on Ceres Controlled by Topography, J. Geophys. Res.-Planets, 125, e2020JE006640, https://doi.org/10.1029/2020JE006640, 2020.
Jónsson, Ó.: Skriðuföll og snjóflóð, Bókaútgafan Norðri, 1, 141 pp., 1957.
Kirk, R. L., Howington-Kraus, E., Rosiek, M. R., Anderson, J. A., Archinal, B. A., Becker, K. J., Cook, D. A., Galuszka, D. M., Geissler, P. E., Hare, T. M., Holmberg, I. M., Keszthelyi, L. P., Redding, B. L., Delamere, W. A., Gallagher, D., Chapel, J. D., Eliason, E. M., King, R., and McEwen, A. S.: Ultrahigh resolution topographic mapping of Mars with MRO HiRISE stereo images: Meter-scale slopes of candidate Phoenix landing sites, J. Geophys. Res.-Planets, 113, E00A24, https://doi.org/10.1029/2007JE003000, 2008.
Kite, E. S. and Conway, S.: Geological evidence for multiple climate transitions on Early Mars. Nat. Geosci. 17, 10–19, https://doi.org/10.1038/s41561-023-01349-2, 2024.
Laskar, J., Correia, A. C. M., Gastineau, M., Joutel, F., Levrard, B., and Robutel, P.: Long term evolution and chaotic diffusion of the insolation quantities of Mars, Icarus, 170, 343–364, https://doi.org/10.1016/j.icarus.2004.04.005, 2004.
Legros, F.: The mobility of long-runout landslides, Eng. Geol., 63, 301–331, https://doi.org/10.1016/S0013-7952(01)00090-4, 2002.
Lucas, A., Mangeney, A., Mège, D., and Bouchut, F.: Influence of the scar geometry on landslide dynamics and deposits: Application to Martian landslides, J. Geophys. Res. Planets, 116, E10001, https://doi.org/10.1029/2011JE003803, 2011.
Lucas, A., Mangeney, A., and Ampuero, J. P.: Frictional velocity-weakening in landslides on Earth and on other planetary bodies, Nat. Commun., 5, 3417, https://doi.org/10.1038/ncomms4417, 2014.
Lucchitta, B. K.: A large landslide on Mars, GSA Bull., 89, 1601–1609, https://doi.org/10.1130/0016-7606(1978)89<1601:ALLOM>2.0.CO;2, 1978.
Lucchitta, B. K.: Landslides in Valles Marineris, Mars, J. Geophys. Res.-Solid, 84, 8097–8113, https://doi.org/10.1029/JB084iB14p08097, 1979.
Lucchitta, B. K.: Valles Marineris, Mars: Wet debris flows and ground ice, Icarus, 72, 411–429, https://doi.org/10.1016/0019-1035(87)90183-7, 1987.
Magnarini, G., Mitchell, T. M., Grindrod, P. M., Goren, L., and Schmitt, H. H.: Longitudinal ridges imparted by high-speed granular flow mechanisms in martian landslides, Nat. Commun., 10, 4711, https://doi.org/10.1038/s41467-019-12734-0, 2019.
Magnarini, G., Mitchell, T. M., Goren, L., Grindrod, P. M., and Browning, J.: Implications of longitudinal ridges for the mechanics of ice-free long runout landslides, Earth Planet. Sc. Lett., 574, 117177, https://doi.org/10.1016/j.epsl.2021.117177, 2021a.
Magnarini, G., Mitchell, T. M., Grindrod, P. M., Schmitt, H. H., and Petro, N. E.: Scaling Relationship Between the Wavelength of Longitudinal Ridges and the Thickness of Long Runout Landslides on the Moon, J. Geophys. Res.-Planets, 126, e2021JE006922, https://doi.org/10.1029/2021JE006922, 2021b.
Magnarini, G., Champagne, A., Morino, C., Beck, C., Philippe, M., and Conway, S. J.: Long Runout Landslides with Longitudinal Ridges in Iceland as Analogues of Martian Landforms [Dataset], Figshare [data set], https://doi.org/10.6084/m9.figshare.22333036.v1, 2023.
Makowska, M., Mège, D., Gueydan, F., and Chéry, J.: Mechanical conditions and modes of paraglacial deep-seated gravitational spreading in Valles Marineris, Mars, Geomorphology, 268, 246–252, https://doi.org/10.1016/j.geomorph.2016.06.011, 2016.
Malin, M.: MRO Context Camera Experiment Data Record Level 0 V1.0, MRO-M-CTX-2-EDR-L0-V1.0, NASA Planetary Data System, https://doi.org/10.17189/1520266, 2007.
Malin, M. C., Bell III, J. F., Cantor, B. A., Caplinger, M. A., Calvin, W. M., Clancy, R. T., Edgett, K. S., Edwards, L., Haberle, R. M., James, P. B., Lee, S. W., Ravine, M. A., Thomas, P. C., and Wolff, M. J.: Context Camera Investigation on board the Mars Reconnaissance Orbiter, J. Geophys. Res.-Planets, 112, E05S04, https://doi.org/10.1029/2006JE002808, 2007.
Mather, A. E., Hartley, A. J., and Griffiths, J. S.: The giant coastal landslides of Northern Chile: Tectonic and climate interactions on a classic convergent plate margin, Earth Planet. Sc. Lett., 388, 249–256, https://doi.org/10.1016/j.epsl.2013.10.019, 2014.
McEwen, A. S.: Mobility of large rock avalanches: Evidence from Valles Marineris, Mars, Geology, 17, 1111–1114, https://doi.org/10.1130/0091-7613(1989)017<1111:MOLRAE>2.3.CO;2, 1989.
McEwen, A. S., Eliason, E. M., Bergstrom, J. W., Bridges, N. T., Hansen, C. J., Delamere, W. A., Grant, J. A., Gulick, V. C., Herkenhoff, K. E., Keszthelyi, L., Kirk, R. L., Mellon, M. T., Squyres, S. W., Thomas, N., and Weitz, C. M.: Mars Reconnaissance Orbiter's High Resolution Imaging Science Experiment (HiRISE), J. Geophys. Res.-Planets, 112, E05S02, https://doi.org/10.1029/2005JE002605, 2007.
McSaveney, M. J.: Chapter 6 – Sherman Glacier Rock Avalanche, Alaska, U.S.A., in: Developments in Geotechnical Engineering, vol. 14, edited by: Voight, B., Elsevier, 197–258, https://doi.org/10.1016/B978-0-444-41507-3.50014-3, 1978.
Mège, D. and Bourgeois, O.: Equatorial glaciations on Mars revealed by gravitational collapse of Valles Marineris wallslopes, Earth Planet. Sc. Lett., 310, 182–191, https://doi.org/10.1016/j.epsl.2011.08.030, 2011.
Melosh, H. J.: Slopes and mass movement, in: Planetary Surface Processes. Cambridge Planetary Science, Cambridge University Press, 319–347, https://doi.org/10.1017/CBO9780511977848, 2011.
Mercier, D., Cossart, E., Decaulne, A., Feuillet, T., Jónsson, H. P., and Sæmundsson, Þ.: The Höfðahólar rock avalanche (sturzström): Chronological constraint of paraglacial landsliding on an Icelandic hillslope, Holocene, 23, 432–446, https://doi.org/10.1177/0959683612463104, 2013.
Mercier, D., Coquin, J., Feuillet, T., Decaulne, A., Cossart, E., Jónsson, H. P., and Sæmundsson, Þ.: Are Icelandic rock-slope failures paraglacial? Age evaluation of seventeen rock-slope failures in the Skagafjörður area, based on geomorphological stacking, radiocarbon dating and tephrochronology, Geomorphology, 296, 45–58, https://doi.org/10.1016/j.geomorph.2017.08.011, 2017.
Molaro, L., Discenza, M. E., Minnillo, M., Komatsu, G., and Miccadei, E.: Absolute dating and evolutionary model of large rock avalanches on Mars: Examples from the Hydraotes Chaos and Tiu Valles region, Icarus, 407, 115778, https://doi.org/10.1016/j.icarus.2023.115778, 2024.
Morino, C., Conway, S. J., Sæmundsson, Þ., Helgason, J. K., Hillier, J., Butcher, F. E. G., Balme, M. R., Jordan, C., and Argles, T.: Molards as an indicator of permafrost degradation and landslide processes, Earth Planet. Sc. Lett., 516, 136–147, https://doi.org/10.1016/j.epsl.2019.03.040, 2019.
Morino, C., Conway, S., Philippe, M., Peignaux, C., Svennevig, K., Lucas, A., Noblet, A., Roberti, G., Butcher, F., and Collins-May, J.: Permafrost molards as an analogue for ejecta-ice interactions at Hale Crater, Mars, Icarus, 391, 115363, https://doi.org/10.1016/j.icarus.2022.115363, 2023.
NASA PDS – Planetary Data System: Welcome to the PDS, https://pds.nasa.gov/ (last access: 31 January 2023), 2024.
Okubo, C. H.: Structural geology of Amazonian-aged layered sedimentary deposits in southwest Candor Chasma, Mars, Icarus, 207, 210–225, https://doi.org/10.1016/j.icarus.2009.11.012, 2010.
Porter, C., Morin, P., Howat, I., Noh, M.-J., Bates, B., Peterman, K., Keesey, S., Schlenk, M., Gardiner, J., Tomko, K., Willis, M., Kelleher, C., Cloutier, M., Husby, E., Foga, S., Nakamura, H., Platson, M., Wethington, M., Williamson, C., Bauer, G., Enos, J., Arnold, G., Kramer, W., Becker, P., Doshi, A., D'Souza, C., Cummens, P., Laurier, F., and Bojesen, M.: ArcticDEM, Harvard Dataverse, https://doi.org/10.7910/DVN/OHHUKH, 2018.
Pudasaini, S. P. and Miller, S. A.: The hypermobility of huge landslides and avalanches, Eng. Geol., 157, 124–132, https://doi.org/10.1016/j.enggeo.2013.01.012, 2013.
Quantin, C., Allemand, P., Mangold, N., and Delacourt, C.: Ages of Valles Marineris (Mars) landslides and implications for canyon history, Icarus, 172, 555–572, https://doi.org/10.1016/j.icarus.2004.06.013, 2004.
Saemundsson, K.: Outlines of the geology of Iceland, Jökull, 29, 7–28, 1980.
Saemundsson, P., Morino, C., and Conway, S. J.: 5.22 – Mass-Movements in Cold and Polar Climates, in: Treatise on Geomorphology, 2nd Edn., edited by: Shroder, J. (Jack) F., Academic Press, Oxford, 350–370, https://doi.org/10.1016/B978-0-12-818234-5.00117-6, 2022.
Schmidt, B. E., Hughson, K. H. G., Chilton, H. T., Scully, J. E. C., Platz, T., Nathues, A., Sizemore, H., Bland, M. T., Byrne, S., Marchi, S., O'Brien, D. P., Schorghofer, N., Hiesinger, H., Jaumann, R., Pasckert, J. H., Lawrence, J. D., Buzckowski, D., Castillo-Rogez, J. C., Sykes, M. V., Schenk, P. M., DeSanctis, M.-C., Mitri, G., Formisano, M., Li, J.-Y., Reddy, V., LeCorre, L., Russell, C. T., and Raymond, C. A.: Geomorphological evidence for ground ice on dwarf planet Ceres, Nat. Geosci., 10, 338–343, https://doi.org/10.1038/ngeo2936, 2017.
Shi, A.-W., Wang, Y.-F., Cheng, Q.-G., Lin, Q.-W., Li, T.-H., and Wünnemann, B.: The largest rock avalanche in China at Iymek, Eastern Pamir, and its spectacular emplacement landscape, Geomorphology, 421, 108521, https://doi.org/10.1016/j.geomorph.2022.108521, 2022.
Shreve, R. L.: Sherman Landslide, Alaska, Science, 154, 1639–1643, https://doi.org/10.1126/science.154.3757.1639, 1966.
Shreve, R. L.: The Blackhawk Landslide, GSA Special papers 108, The Geological Society of America, Boulder, Colorado, 47 pp., https://doi.org/10.1130/SPE108, 1968.
Singer, K. N., McKinnon, W. B., Schenk, P. M., and Moore, J. M.: Massive ice avalanches on Iapetus mobilized by friction reduction during flash heating, Nat. Geosci., 5, 574–578, https://doi.org/10.1038/ngeo1526, 2012.
Smith, D. E., Zuber, M. T., Solomon, S. C., Phillips, R. J., Head, J. W., Garvin, J. B., Banerdt, W. B., Muhleman, D. O., Pettengill, G. H., Neumann, G. A., Lemoine, F. G., Abshire, J. B., Aharonson, O., David, C., Brown, Hauck, S. A., Ivanov, A. B., McGovern, P. J., Zwally, H. J., and Duxbury, T. C.: The Global Topography of Mars and Implications for Surface Evolution, Science, 284, 1495–1503, https://doi.org/10.1126/science.284.5419.1495, 1999.
Thordarson, T. and Höskuldsson, Á.: Iceland: Classic Geology in Europe 3, in: 3rd Edn., Dunedin Academic Press, 280 pp., ISBN 10:1780460929 ISBN 13:978-1780460925,2022.
Vardoulakis, I.: Catastrophic landslides due to frictional heating of the failure plane, Mech. Cohes.-Frict. Mater., 5, 443–467, https://doi.org/10.1002/1099-1484(200008)5:6<443::AID-CFM104>3.0.CO;2-W, 2000.
Vick, L. M., Mikkelsen, M., Corner, G. D., Kjellman, S. E., Trønnes, L., Hormes, A., Allaart, L., and Bergh, S. G.: Evolution and temporal constraints of a multiphase postglacial rock slope failure, Geomorphology, 398, 108069, https://doi.org/10.1016/j.geomorph.2021.108069, 2021.
Voight, B. and Faust, C.: Frictional heat and strength loss in some rapid landslides, Géotechnique, 32, 43–54, https://doi.org/10.1680/geot.1982.32.1.43, 1982.
Watkins, J. A., Ehlmann, B. L., and Yin, A.: Long-runout landslides and the long-lasting effects of early water activity on Mars, Geology, 43, 107–110, https://doi.org/10.1130/G36215.1, 2015.
Weidinger, J. T., Korup, O., Munack, H., Altenberger, U., Dunning, S. A., Tippelt, G., and Lottermoser, W.: Giant rockslides from the inside, Earth Planet. Sc. Lett., 389, 62–73, https://doi.org/10.1016/j.epsl.2013.12.017, 2014.
Whalley, W. B., Douglas, G. R., and Jonsson, A.: The Magnitude and Frequency of Large Rockslides in Iceland in the Postglacial, Geogr. Ann. A, 65, 99–110, https://doi.org/10.2307/520724, 1983.
Wyrwoll, K. H.: Causes of rock-slope failure in a cold area: Labrador-Ungava., in: Reviews in Engineering Geology: Vol. 3 – Landslides, Geological Society of America, 59–67, https://doi.org/10.1130/REG3-p57, 1977.
Zuber, M. T., Smith, D. E., Solomon, S. C., Muhleman, D. O., Head, J. W., Garvin, J. B., Abshire, J. B., and Bufton, J. L.: The Mars Observer laser altimeter investigation, J. Geophys. Res.-Planets, 97, 7781–7797, https://doi.org/10.1029/92JE00341, 1992.
- Abstract
- Introduction
- Data and methods
- Landslides in Iceland
- Landslides on Mars
- Morphometric and morphological comparison between Icelandic and Martian long-runout landslides with longitudinal ridges
- Conclusions
- Data availability
- Author contributions
- Competing interests
- Disclaimer
- Special issue statement
- Acknowledgements
- Financial support
- Review statement
- References
- Supplement
- Abstract
- Introduction
- Data and methods
- Landslides in Iceland
- Landslides on Mars
- Morphometric and morphological comparison between Icelandic and Martian long-runout landslides with longitudinal ridges
- Conclusions
- Data availability
- Author contributions
- Competing interests
- Disclaimer
- Special issue statement
- Acknowledgements
- Financial support
- Review statement
- References
- Supplement